Before about 200 My ABB.1After Big Bang, only simple atoms existed, mostly single protons (hydrogen) and a small number of proton-neutron pairs (helium).They formed clouds of particles which are referred to by the Latin word, nebulae. There are nebulae out there today, but their composition has changed since the first stars started to form. We will see how.
The life cycle of stars follows a series of steps.
Formation of protostars
After about 200 My, the universe had cooled enough that the gravitational force could finally come into play, so particles of matter were attracted to each other. Dust particles stuck together and formed tiny clumps which in turn adhered to orm larger ones. Thus, a larger clump of gaseous matter was built up. As its density increased, it became warmer. If the mass of such a clump exceeds the so-called Jeans mass2The Jeans mass depends on temperature and density, but is typically many thousands of solar masses., the matter composing it becomes so dense that any generated radiation (photons) cannot escape, so the clump becomes opaque. The trapped photons cause the clump to heat even faster. The clump had become a protostar.
So first, the strong force formed nuclei, after which the electromagnetic force formed atoms. Then the gravitational force formed clusters of matter which became stars, galaxies, planets and black holes.
The opposing forces of pressure and gravity have opposite thermodynamic effects. Gravity pulls matter into a more organized state, so one of lower entropy. However, the radiation from the outward pressure disperses more than enough energy into space to guarantee a global increase in entropy.
A protostar may or may not go on to become a star. If its mass is too low (<8% of the mass of our Sun), its temperature never becomes high enough for fusion to begin and the protostar becomes what astronomers call a brown dwarf.
Main-sequence stars
In larger protostars, when the temperature rises to 107 Kelvin, a series of nuclear fusion reactions referred to as the proton-proton chain uses protons to produce helium. First, the protons fuse together to form deuterium (2H, with one proton and one neutron in its nucleus), then 3He (2 protons and one neutron) and finally 4He (2 protons and two neutrons).3A word on notation. A superscript before an element’s symbol denotes its atomic mass, essentially the total number of neutrons plus protons in the nucleus. When fusion starts to take place, the protostar has becomes a star. This first occurred only when the universe was some 200 million years or more old.
The fusion process is often referred to as “burning” of hydrogen, but it has nothing to do with ordinary terrestrial burning, or oxidation. It is really 4 protons forming 2 protons and 2 neutrons held together to form a helium nucleus. Since the nucleus weighs somewhat less than the initial 4 protons, the extra mass is converted into a great deal of energy4If only we could harness this fusion energy here on Earth! due to the large size of the speed of light, c, in the famous equation from GR,
E = mc2.
Some of this energy is emitted in the form of light emitted by the the stars, so from the moment fusion began, the universe was not only transparent, there was light (i.e., photons) in it.
Astronomers visualize the formation and subsequent life of stars by a two-dimensional plot called the Hertzsprung-Russell diagram (hereafter abbreviated HR). The HR is somewhat analogous to the Periodic Table of the elements in that it arranges stars by their properties in such a way as to show similarities between them. It is a scatter plot (points in a 2-dimensional space) of luminosity (or brightness, on the Y-axis) versus temperature (or spectral class, on the X-axis). Stars close to one another on the diagram are found to share many properties. As stars evolve, they move from one point to another on the diagram, unlike elements, which maintain their place in the periodic table. About 90% of stars fall on the diagonal – called the main sequence – between hot, luminous stars in the upper-left-hand corner and cool, dim ones in the lower-right-hand corner. Our Sun lies near the center of the main sequence – for the moment (where the “moment” will last about another 5 Gy). A star’s position in the main sequence is mainly a function of its mass. The current state of the Sun is taken as the standard luminosity.
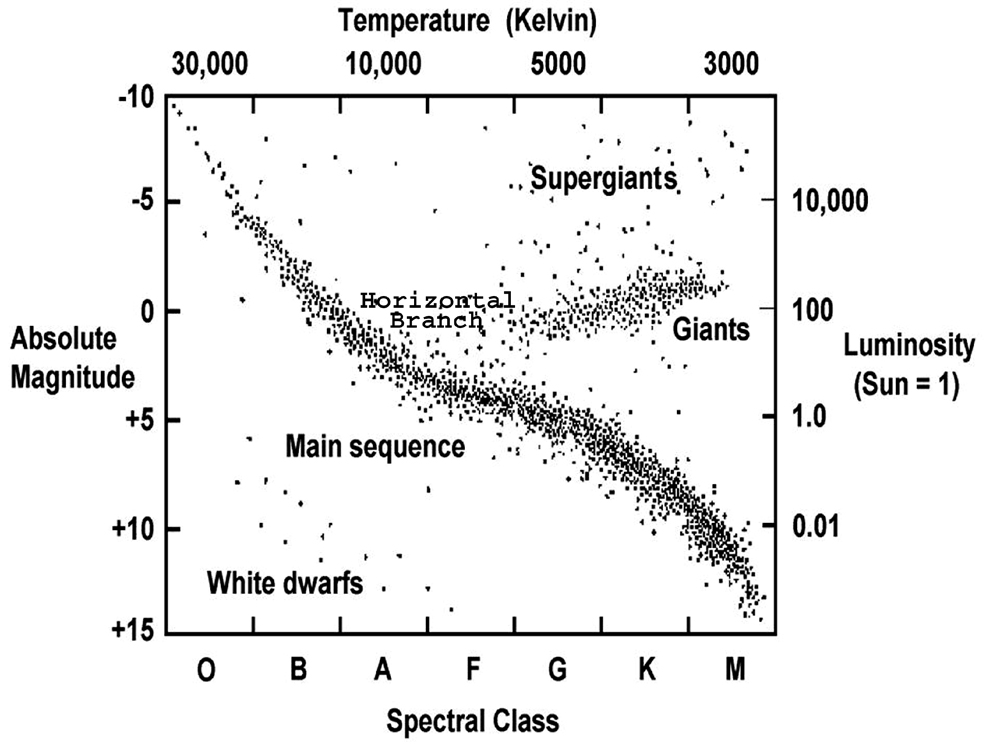
Hertzsprung-Russell Diagram, Chandra X-ray Observatory via NASA
Red giant phase
A star is maintained by the so-called hydrostatic equilibrium between the outwardly-directed pressure due to the fusion in the core and the inwardly-directed force of gravity due to the star’s mass. Larger stars burn faster and so have briefer lives than smaller stars. But in all stars, the hydrogen fuel in the core is eventually depleted. The pressure then is reduced to where it can no longer counteract the force of gravity and the core contracts. As it does so, it heats up and generates increasing outward pressure. If the star is very small, it just radiates the energy away. But for most stars, there is still some hydrogen in a thin layer on the outside of the core and the core-generated heat causes it to begin to fuse into helium in hydrogen shell fusion, as opposed to the hydrogen core fusion which has taken place until now. Observation of the envelope, which is all we can see, will first show a slight cooling with only a small increase in brightness, but then the increased pressure from the core will bring about an increase in size of a hundredfold or more at approximately constant temperature. This is shown on the HR diagram below. The star’s color will change to orange and it will become a red giant. When the Sun reaches this stage, it will probably engulf the inner four planets – among them, Earth.
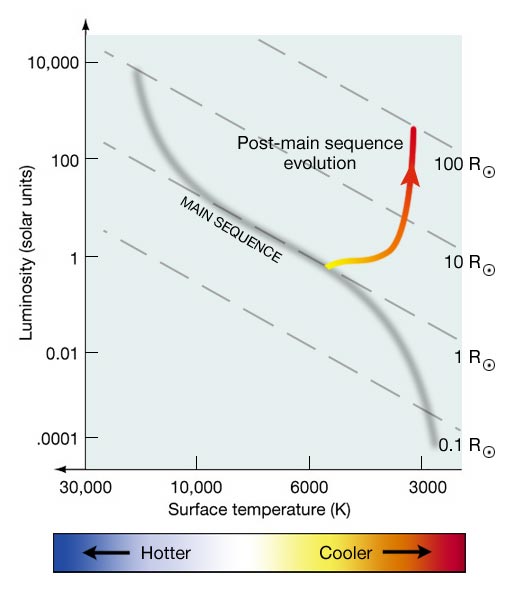
Evolutionary track for a Sun-like star to the red giant phase, Creative Commons image from Pennsylvania State University
After the red-giant phase, what happens depends on the mass of the star. We will mention a few possibilities. For the moment, let us go on considering a star the size of the Sun.
Triple-alpha or red-supergiant phase
The star’s core still is not in equilibrium and continues to contract until it hits the limit imposed by the QM exclusion principle, which keeps its electrons from occupying the same QM state. Within the core, the helium resulting from the preceding hydrogen core fusion begins to fuse into heavier elements,8Be, 12C (and 16O, in still heavier stars). Since 12C formation requires three alpha particles (4He nuclei), the process is called the triple-alpha process and the star is in the core helium fusion phase. The star now has two layers, the inner one fusing to heavier elements than the outer one.
For the Sun, this stage will last less than about 1 billion years, during which it will “burn” 4He into 12C and 16O. A star of about this size will live for a total of around 10 Gy5Most authors would say 10 billion years, but in order to be consistent with later chapters, we will say 10 Gy. Since the Sun has already lived for almost 5 Gy (as we shall see in the geology chapter), it has (and we have) about 5 Gy left.
The core helium is used up faster than its hydrogen was and when this occurs, the core contracts again. The increased temperature causes a thin shell of remaining helium just inside the hyrdogen-fusion shell to go on fusing. The core now has three layers: an inner layer of carbon and oxygen, a middle layer of helium fusion and an outer shell of hydrogen fusion. The star expands again and becomes a red supergiant.
Nebula phase
After this, the loss of mass to solar wind and the reduced supply of nuclear fuel will lower the star’s pressure to where gravity shrinks it to approximately the size of the Earth. Its outer layers will be cast outwards into an expanding shell of gas and for a while it will be a hot core of carbon surrounded by a planetary nebula (cloud) of ionized gas.6Note that a planetary nebula has nothing to do with planets. UV radiation from the cooling core lights up the gaseous nebula.
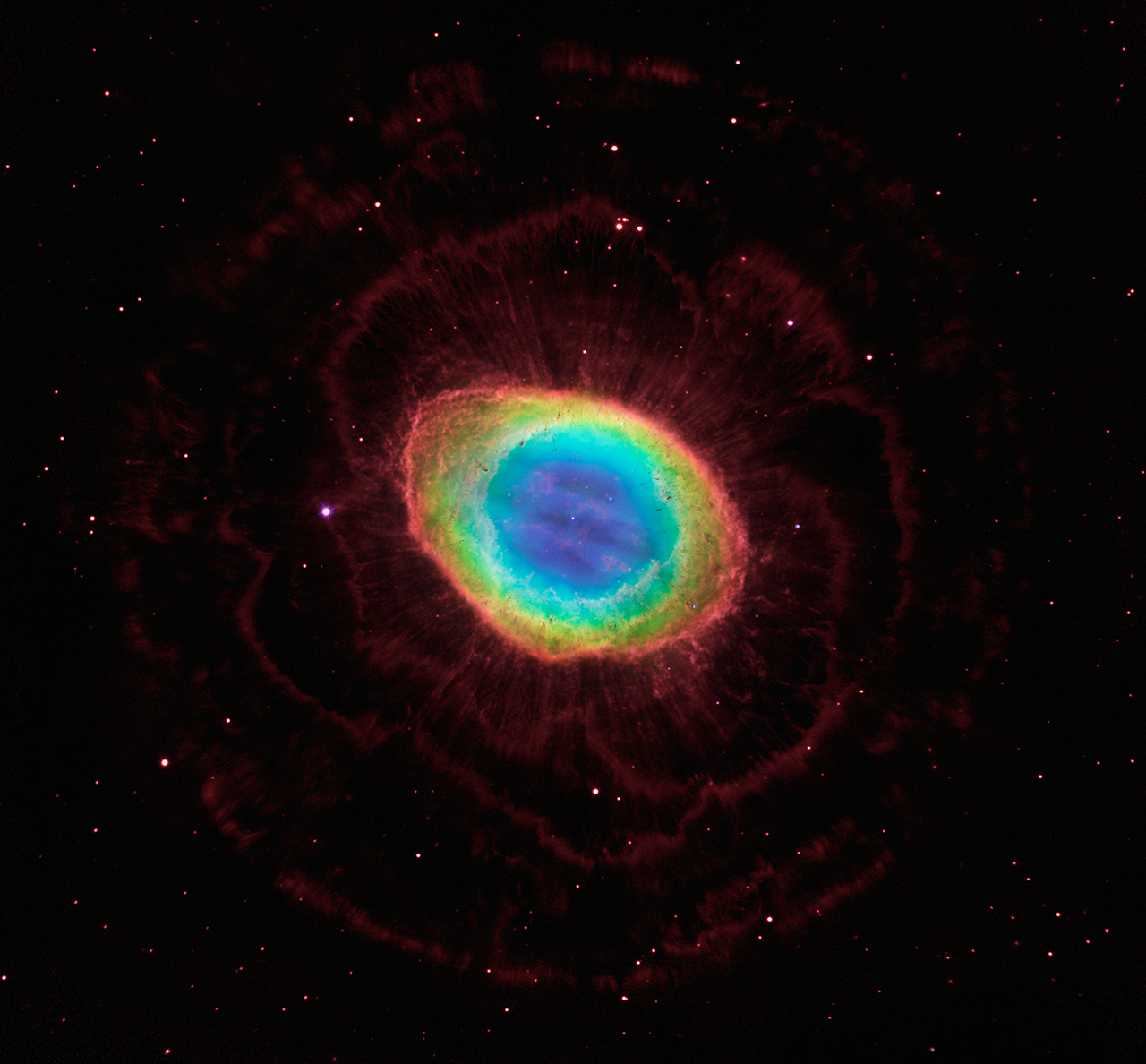
The Ring nebula, M57, NGC 6720, from NASA
After the dust drifts away, all that will be left of the Sun’s core will be a relatively cool and invisible white dwarf. The star is supported against collapse only by the QM exclusion principle. Eventually, the white dwarf will cool into a hunk of cold iron – an unglamorous end to quite a stellar career. This will be the fate of any star less than about 1.4 times the mass of the Sun, a figure known as the Chandrasekhar limit.
One more time…
Within the matter ejected into space as nebula, the whole process can start over again with agglomeration of dust to form protostars, but with two important differences.
- The nebula dust now contains heavier elements like carbon and oxygen.
- Exploding stars, in particular, the supernovae to be discussed shortly, send out shock waves which may provoke the formation of new protostars.
Variable stars – cepheids
The main-sequence expansion to a red giant does not necessarily go smoothly. Some stars will be in unstable equilibrium, like a wobbly tight-wire walker. Pressure may cause them to overshoot and become too big, then gravity will take over and they may become too small. And so on, back and forth. Such stars are called either Cephiad variables or RR Lyrai variables.
Massive stars
For stars distinctly more massive than the Sun, the sequence may go further. In a later stage, 12C and 16O may fuse to 20Ne, 23Na, 24Mg and 28Si. In turn, 28Si may fuse to 56Fe, but this can only take place in the most massive stars. In any case, that is where the process stops; Fe does not fuse to anything else. This is the second step of nucleosynthesis.
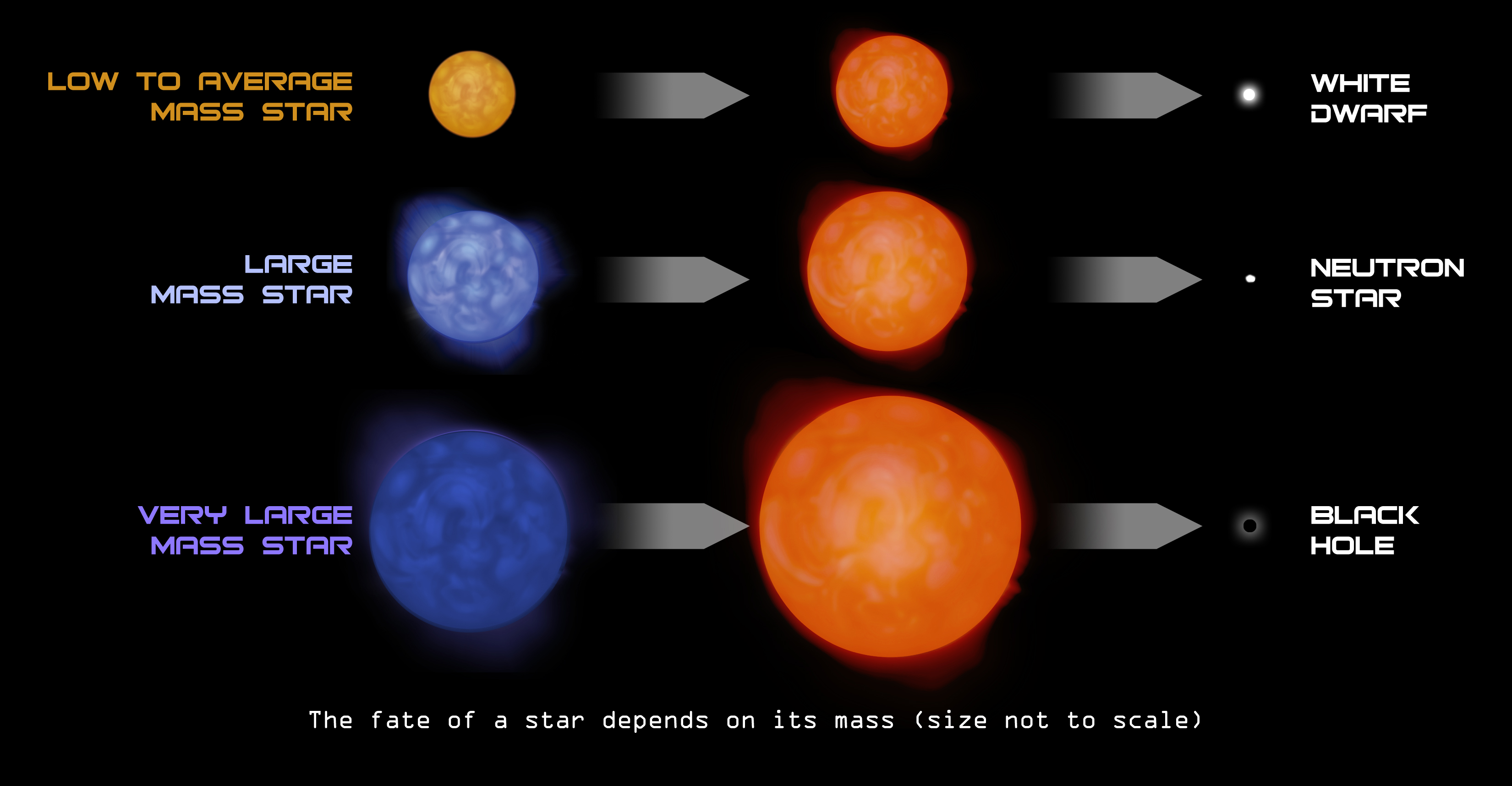
The fate of a star depends on its mass, from Chandra X-ray Observatory, via NASA/CXC/SAO.
As massive stars pass from step to step of the fusion process, burning successively carbon and oxygen, then other elements, each step takes place in the core’s center, pushing the previous step’s reactants outwards. So the star comes to have a number of layers, like an onion, with different fusion reactions taking place in different layers. Each successive step takes less time, the fusion of Si into Fe taking only on the order of four days. Once the Si is converted to Fe, though, the pressure drops and nothing can keep the star from collapsing. The outer layers “drop” towards the center and bounce off the core, causing the whole structure to explode and become a Type II supernova (SNII). Such an enormous amount of energy is released that the light is as bright as that as of several billion Suns. For some days or weeks, it may be the brightest object in the sky, depending on how far away it is. Light from the supernova gets brighter over a period of about 20 days and then decreases slowly over a year or so.
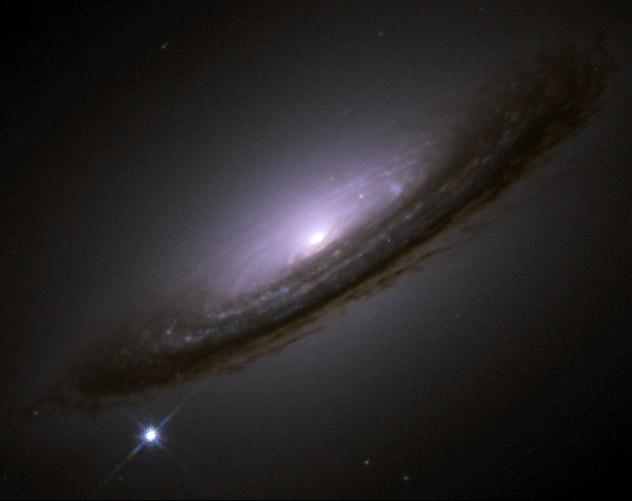
Supernova 1994D, NGC 4526 from Hubble Gallery
By this time, many neutrons have been created by the combination of electrons and protons and some of them react with the Fe to create heavier, radioactive elements which subsequently are expelled into the supernova. These radioactive elements then decay with emission of gamma rays. (It has been proposed that such gamma rays from a huge supernova may have been the cause of the Ordovician-Silurian extinction around 447-443 Mya; see the geology chapter.) Be that as it may, many of these atoms combine by simple chemical reactions and form a new, but richer, dust cloud in space, richer because it contains all those heavier elements produced by the first-generation stars. These elements include C and O which are necessary for life as we know it. This cloud may be the source for new stars. This is the third and final step in nucleosynthesis.
To resume, nucleosynthesis takes place in three steps:
- In Big-Bang nucleosynthesis, the lightest atoms, H, He and Li are formed.
- In stellar fusion, heavier elements are forged, through C, O and up to Fe.
- In supernovae, still heavier elements are formed, including radioactive ones.
The remaining core of the exploded star is reduced to a small, extraordinarily dense clump of neutrons. Such neutron stars often rotate rapidly and have strong magnetic fields. Jets of particles are expelled along the poles and appear to flash as the star spins. Such start are called pulsars.
However, if the collapsed core outweighs three solar masses, gravity is so strong that nothing can escape it, not even light. The core becomes a black hole.
Expansion of space – Type I supernovae
Many – maybe most – stars in the universe are binary, meaning that two relatively nearby stars revolve around the center of gravity of the pair. If one of them is below the Chandrasekhar limit and so becomes a white dwarf, it may pick up matter radiated by its twin. If enough such matter is accreted to its surface, it may become massive enough, compared to the Chandrasekhar limit, that the electron degeneracy7Another name for the Exclusion Principal can no longer support it and it explodes into a Type Ia supernova (SNIa). Such objects are extraordinarily bright.8The most recently viewed one in our galaxy was observed in 1572 by the young Danish astronomer Tycho Brahe. They are important to cosmologists as they all burn with the same luminosity, making them standard “candles” which can be used to estimate their distance from the Earth (by their apparent brightness) and their speed (from the Doppler shift of their spectra, known as the red shift). In this way, astronomers have measured the speed and distance of galaxies and made an extraordinary discovery: The expansion of space has been accelerating for the last 7 billion years.
Stars are important, but there are larger and smaller structures in the universe. We live on one of them. So on to galaxies, clusters and large-scale structures.
Notes
↑1 | After Big Bang |
---|---|
↑2 | The Jeans mass depends on temperature and density, but is typically many thousands of solar masses. |
↑3 | A word on notation. A superscript before an element’s symbol denotes its atomic mass, essentially the total number of neutrons plus protons in the nucleus. |
↑4 | If only we could harness this fusion energy here on Earth! |
↑5 | Most authors would say 10 billion years, but in order to be consistent with later chapters, we will say 10 Gy |
↑6 | Note that a planetary nebula has nothing to do with planets. |
↑7 | Another name for the Exclusion Principal |
↑8 | The most recently viewed one in our galaxy was observed in 1572 by the young Danish astronomer Tycho Brahe. |