What developmental biology tells us
Knowing about embryonic development is especially helpful in understanding the structure of the brain.
Fertilization, pre-embryonic development
Human pre-natal development traverses three stages:
- The first two weeks of human development are called the pre-embryonic stage;
- weeks 3-8, embryonic;
- and after that, fetal.
Let’s start at the beginning.
Of the tens of millions of human sperm ejected during intercourse, millions are destroyed by the acid environment (pH 3.8) of the vagina or trapped by gluey cervical mucus. Thousands more are killed by uterine leukocytes.1Only strong sperm will make it. Force of numbers helps, too. The sperm follow the gradient of the chemical progesterone, which is released by the oocyte (egg). The surviving sperm usually meet the oocyte in the uterine (fallopian) tubes between the ovary and the uterus, since an unfertilized egg cannot live long enough to make the three-day journey along the tube. It is a question of force of numbers, as hundreds of sperm burrow through the oocyte’s outer protective cover, the corona radiata. Together, they release digestive enzymes from their tips to degrade both this layer and the inner layer, the zona pellucida. Finally, one sperm may manage to fuse its cell membrane with that of the oocyte so that it can release its DNA-containing nucleus into the oocyte. Then the work of the sperm is finished, as there exist mechanisms to prevent more than one sperm from fertilizing the oocyte. The process by which the haploid oocyte and the haploid sperm form a diploid zygote is rather more complicated than this, but that is the result.
Some time after fertilization, all the male mitochondria in the embryo are destroyed, leaving only the maternal mitochondria. It is not known how male mitochondria are recognized, but a gene has been identified, cps-6, which is imported into the male mitochondria and breaks them down. Since suppression of this gene leads to increased mortality in these embryos, there is certainly an evolutionary cause for the destruction, but it is not yet known.2How paternal mitochondria are destroyed in an embryo”, https://whyevolutionistrue.wordpress.com/2016/06/24/paternal-mitochondria-are-destroyed-in-an-embryo/.
The conceptus, the zygote and its containing membranes, takes three days to reach the uterus. Along the way, mitotic cleavage increases the number of cells, called blastomeres, but without increasing the total overall size. By the time the conceptus reaches the uterus, it is composed of sixteen compacted cells, together called a morula. Mitosis continues, soon making about 100 cells arranged in a dense shell around a fluid-filled cavity called a blastocoel. The conceptus is now called a blastocyst. The cells of the outer shell are called trophoblasts and will form structures like the placenta.. Within the cavity, other cells form an inner cell mass which will become the embryo.3Information in these paragraphs on fertilization comes mainly from Wolpert (2011) and Openstax Anatomy and Physiology, http://cnx.org/contents/FPtK1zmh@8.25:FwQJfRAS@3/Embryonic-Development.
During this time, the inner mass is composed of totipotent, or pluripotent, stem cells, which can differentiate to form any type of cell.
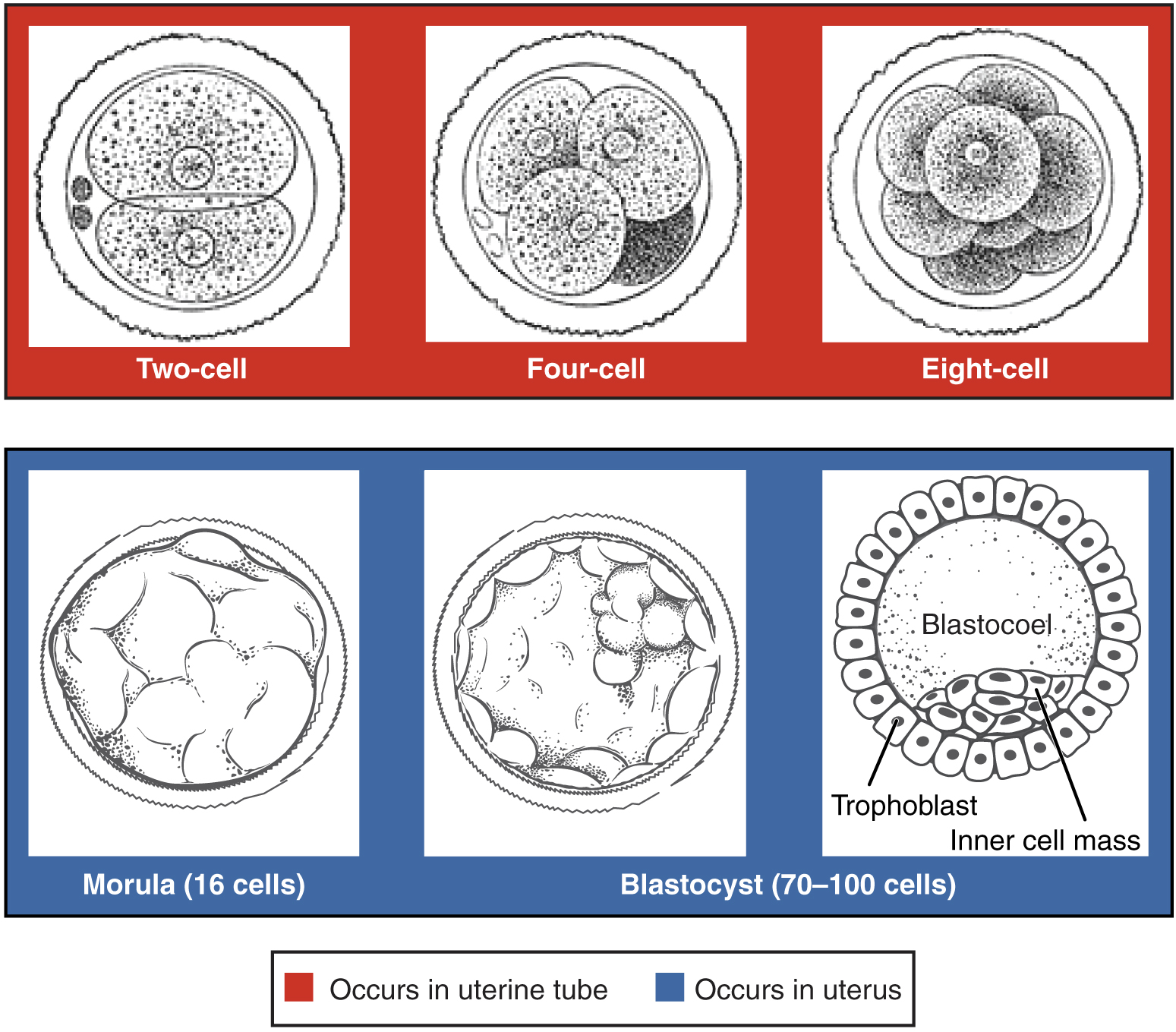
Pre-embyronic cleavages, from Openstax College
After a week, if all goes well, the blastocyst will attach to the inner uterine wall, the endometrium. In over half the cases, though, all does not go well and the blastocyst goes out with the menses. In successful implantation, the blastocyst eats through the endometrium, which then reforms so as to surround the embryo. The implantation marks the end of the pre-embryonic and the start of the embryonic state.
At the second week of embryonic development, the human zygote has developed into a two-layered embryonic disc contained between the blastocyst cavity (which will become the yolk sac) and the amniotic cavity. The ventral (forward) layer against the blastocyst cavity is the hypoblast. The dorsal layer against the amniotic cavity is the epiblast.
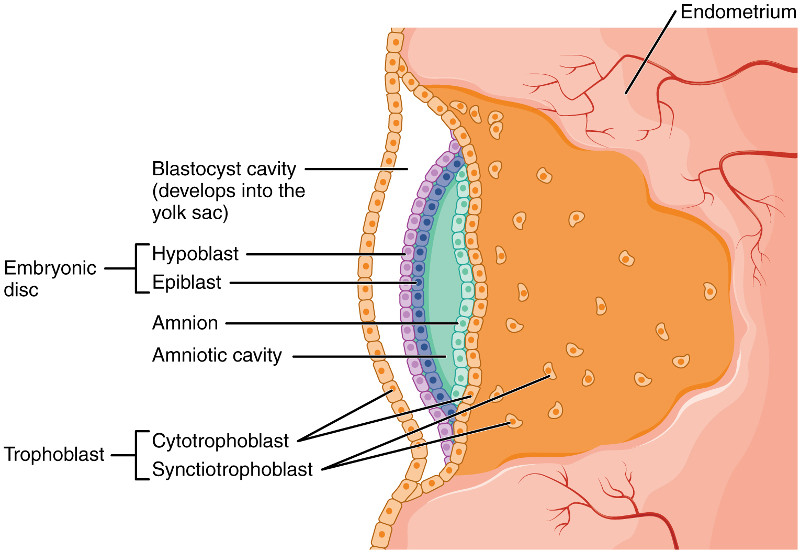
Human embryo before gastrulation, after Openstax College
Gastrulation and embryogenesis – germ layer formation
At the third week, gastrulation takes place, converting the embryo from a two-dimensional disc into a complex three-dimensional structure formed of three germ layers. An indentation called the primitive streak forms along the dorsal (toward the back) surface of the epiblast. Growth factors produced by cells at the caudal (toward the posterior) end of the streak bring about cell multiplication. New cells migrate to the surface of the epiblast where they are specified as one of two new types, then migrate through the streak and form two new cell layers called germ layers.
- One layer, called the endoderm, pushes aside the hypoblast and comes to lie against the yolk sac. The endoderm will form the epithelial lining of the internal organs, among other things.
- A middle layer called the mesoderm is formed next to that. It will form the skeleton, muscles and connective tissues.
- The remaining epiblast cells become the third germ layer, the ectoderm. It will form the integumentary (skin) and nervous systems.
In chordates (such as us), as the neural streak shortens and disappears, it leaves behind a rod-like structure called the notochord. The notochord will later become the nucleus pulposus, the viscous inner core of the inter-vertebral discs that can cause such excruciating back pain when they function badly.
The mesoderm along the notochord forms paired lateral bulges called somites. Positional identity along this axis is furnished by our old friends the hox genes. As we have seen, these genes are expressed in the same order in the DNA as along the antero-posterior axis of the developing embryo. From the somites will develop the spinal column and its 33 vertebrae, as well as associated skeletal muscle.
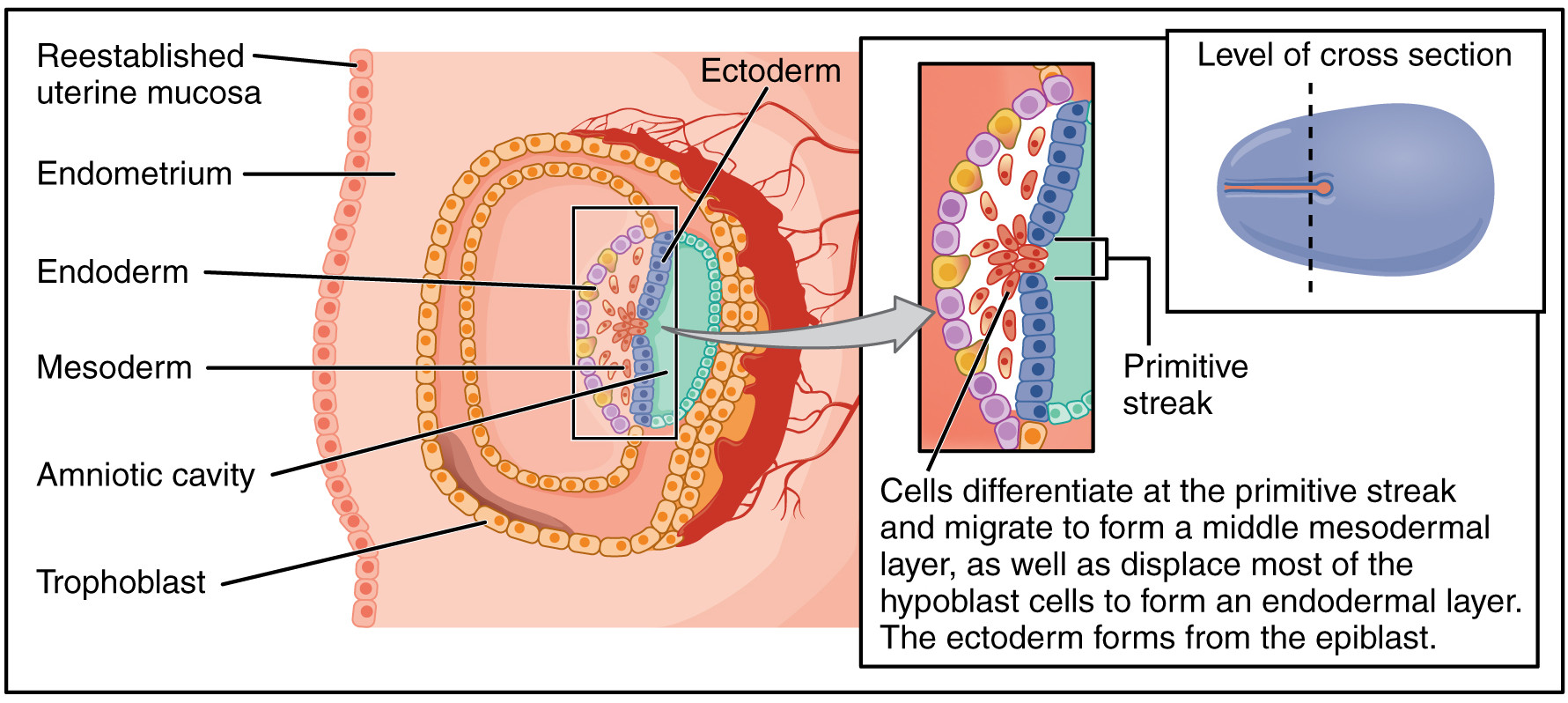
Gastrulation of the human embryo, after Openstax College
Three mechanisms are important for these changes:
- Changes in cell shape are important in gastrulation and other processes. For instance, if a cell contracts on one side, it will become wedge-shaped. Joined to other cells with the same shape, an arc is produced, which is how a flat sheet of cells can bend. Such changes originate in the cytoskeleton.
- Cells have proteins on their surfaces which bind selectively to proteins on other cells, thus causing specific cells to adhere together. Different adhesive molecules cause different cells to adhere to one another.
- Cells can move relative to other cells by extending a long process called a filopodia, which is pushed out by elements of the cell cytoskeleton. The filopodia then move by a retraction mechanism similar to the one used by muscles to contract.
Neurulation – beginning of organogenesis and the nervous system
Soon after gastrulation, the rudiments of the CNS develop in the process of neurulation. Some cells of the ectoderm differentiate into neurepithelium and form a thickened area of the dorsal ectoderm called the neural plate. As shown in the figure, cells in the plate change shape and form a groove, the neural groove. The sides of the groove constitute the neural folds, which invaginate (grow out and come together to form a depression) and fuse, forming a neural tube. Cells along the tube change their adhesive properties so that the tube separates from the plate. The entire CNS will develop from the neural tube walls. The posterior part of the neural tube is formed differently, from a rod of cells which develop an interior cavity.
As the neural folds fuse, some ectoderm at the edges of the neural plate is squeezed off and is then situated between the neural tube and the ectoderm. This is called the neural crest and is the source of the entire PNS.
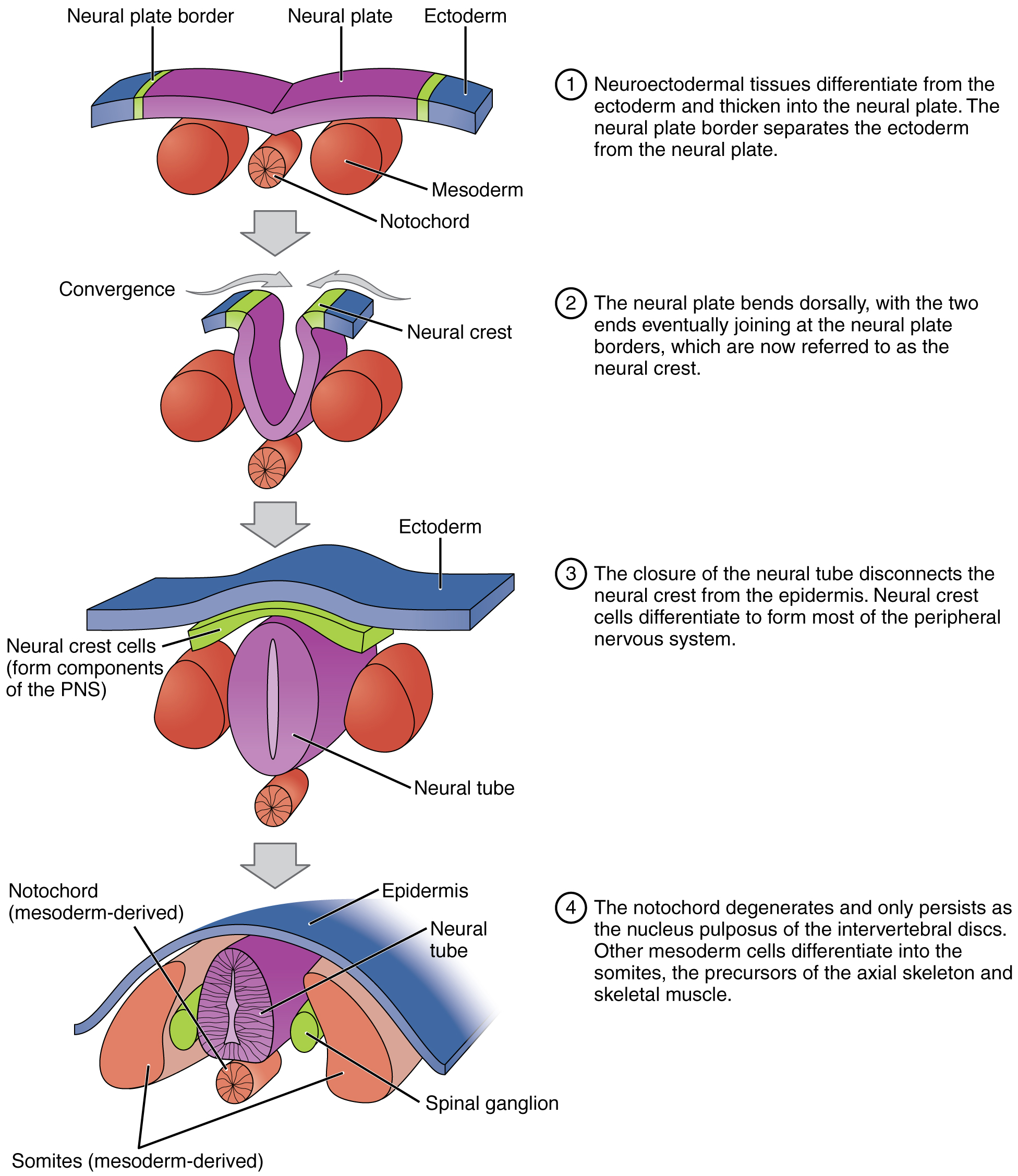
Neurulation forms the rudiments of the CNS and skeleton, from Openstax College
Organogenesis
By eight weeks after conception, the rudiments of all the basic organs have been set up. This is called organogenesis. Neurulation is the first important stage of organogenesis.
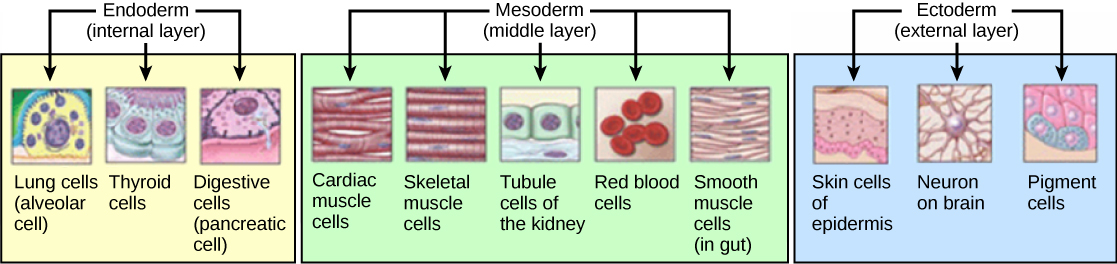
Development of the germ layers in development, from Openstax College
The three germ layers develop into different tissues as shown in the figure. Not shown are the development of bones from the mesoderm, or the development of the CNS from the ectoderm.
The following table shows a brief outline of embryonic development.
Week | Major events |
4-5 | The heart forms first as a tube, like the CNS, and starts beating around the beginning of week four. The liver starts to produce blood cells, a process which will later pass to bone marrow. Eye pits, limb buds and the beginning of the pulmonary system form. |
6 | Limb development starts in earnest. |
7 | Nostrils, outer ears and lenses form. |
8 | The major brain structures are in place. External genitalia are visible, but male and female are indistinguishable. Bone starts to take over from cartilage. |
As it grows, the embryo folds so as to take on the familiar cylindrical shape, pointed at on end, with a primitive gut tube surrounded by the endoderm.
The organism is now at the stage of fetal development. From this point, we will restrict our interest to the consideration of nervous-system development.
Development of the nervous system
Some knowledge of the development of the nervous system is essential to understanding the structure of the brain.
The anterior neural tube develops into the brain. It is first differentiated into three sac-like primary vesicules, from front to back:
- the prosencephalon (forebrain)
- the mesencephalon (midbrain)
- the rhombencephalon (hindbrain), connected to the caudal neural tube.
As the prosencephalon begins to differentiate, two pairs of secondary vesicles grow on either side:
- the optic vesicles, which will eventually become the optic nerves and the retinas (so the retina is part of the brain); and
- the telencephalic vesicles, or telencephalon.
The remaining unpaired part of the prosencephalon is called the diencephalon, or “between brain”. The telencephalic vesicles grow out, then bend back over the diencephalon. From them grow another pair of vesicles, the olfactory bulbs. Cells within the telencephalon develop, forming new structures and axon systems. Spaces remain between the sides of the telencephalon and the diencephalon; they will fill with cerebrospinal fluid (CSF) and become the ventricles of the brain. The neurons within the gray matter of the telencephalon form the cerebral cortex and the basal telencephalon. The diencephalon differentiates into the thalamus and the hypothalamus. Axons from the developing forebrain extend to connect with other parts of the nervous system.4This brief summary is based on Bear, 178-192.
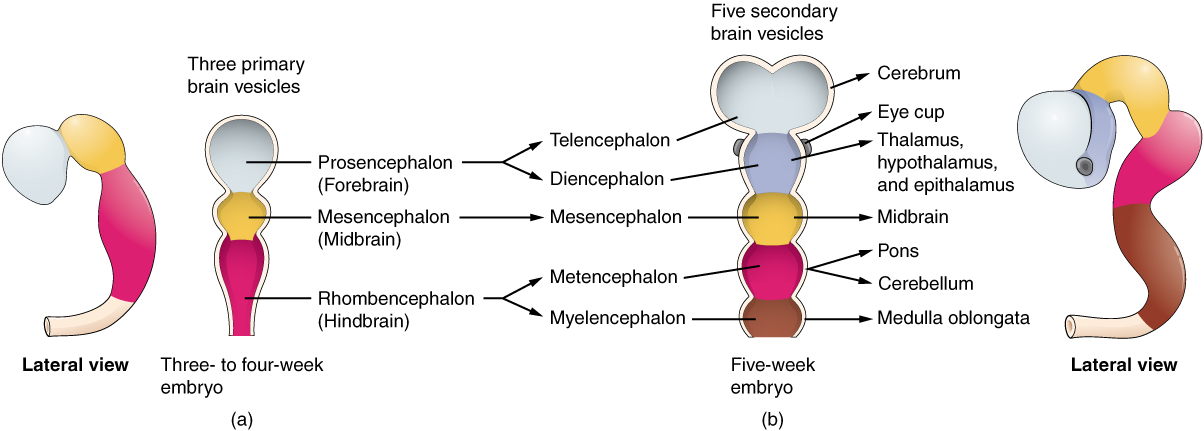
Brain vesicle development, from Openstax College
The hindbrain develops into the pons, medulla oblongata and cerebellum. In a coronal (side to side) section of the brain, it is easy to see how the telencephalon has grown down laterally to the diencephalon. The ventricles are also visible as dark areas.
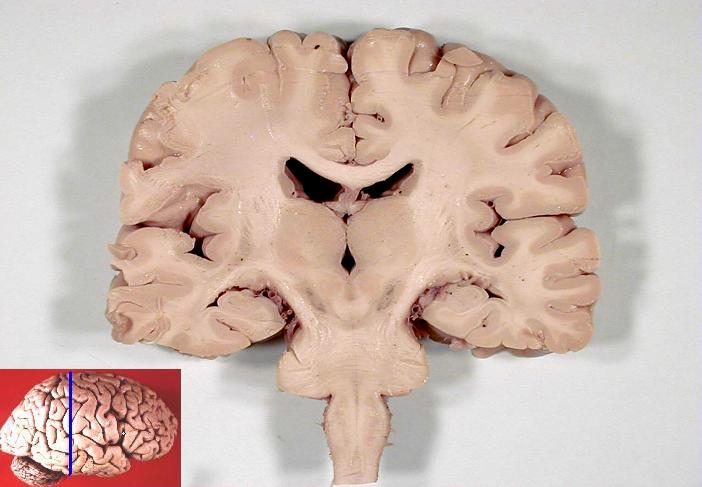
Coronal section of human brain5Looks a bit like the Hulk, doesn’t it?, from John A Beal, via Wikimedia Commons
Next, let’s go to the next subject, the central and peripheral nervous systems.
Notes
↑1 | Only strong sperm will make it. Force of numbers helps, too. |
---|---|
↑2 | How paternal mitochondria are destroyed in an embryo”, https://whyevolutionistrue.wordpress.com/2016/06/24/paternal-mitochondria-are-destroyed-in-an-embryo/. |
↑3 | Information in these paragraphs on fertilization comes mainly from Wolpert (2011) and Openstax Anatomy and Physiology, http://cnx.org/contents/FPtK1zmh@8.25:FwQJfRAS@3/Embryonic-Development. |
↑4 | This brief summary is based on Bear, 178-192. |
↑5 | Looks a bit like the Hulk, doesn’t it? |