The Archean Eon – appearance of life
The period from about 3.8 to 2.5 Gya is referred to as the Archean Eon.
Geology and atmosphere
Over the period of about 3.2-2.7 Gya, rocks on the surface came together to form the first cratons, which would become the central cores of continental plates. Sediments from the eon, which indicate that the rock cycle (volcanism-sedimentation-metamorphism) was in action, provide evidence for the existence of continents and oceans. The oldest existing continental rocks date from the Archean at about 4 Gya. By the end of the Archean, plate tectonics was under way.
Solar energy received at the surface of the Earth was about 20 to 25 % lower than present, which could have made the planet too cold for life to be established, but the CO2 retained heat beneath the atmospheric layer, causing a greenhouse effect which slowly raised atmospheric temperatures. Sunlight striking the water vapor caused photochemical dissociation, the breaking up of the water molecules and the bonding together of the resulting oxygen atoms to create ozone, or O3. In time, the ozone came to protect the surface of the Earth from ultraviolet radiation from the sun. At the same time, it prevented further chemical dissociation, which therefore has not played an important role in the oxygenation of the atmosphere. Further increase of atmospheric oxygen had to wait for photosynthesis, as described below.
Life and atmosphere
Arguments over what was the earliest form of life (and who discovered it) probably are not over yet. Currently, the oldest fossils would be of bacteria from Australia, dating from 3.4 Gya. What makes them interesting is the fact that their metabolism was based on sulfur rather than oxygen, which was not yet common in the atmosphere.
For comparison, the oldest fossil evidence for cyanobacteria dates from 2.22 Gya; for eukaryotes, 1.78-1.68 Gya.
Life may be defined as “… a self-sustaining chemical system capable of incorporating novelty and undergoing Darwinian evolution.” There are several hypotheses about its origin on Earth, especially
- the “primordial soup” hypothesis,
- the hydrothermal vent hypothesis
- the volcanic pool hypothesis.
The “primordial soup” hypothesis considers life to have been brought about using energy from electricity (lightning) in a mixture of gases including water and methane. Such production of organic molecules has been demonstrated in the laboratory, but fails to convince many scientists because it depends greatly on the composition of the atmosphere at the time. In particular, it is now thought that CO2 was far more prevalent than methane. More recent experiments with different mixtures have produced similar organic compounds. The discovery of amino acids on meteorites adds weight to the hypothesis that varying atmospheric conditions could lead to production of organic molecules.
The hydrothermal vent hypothesis exists in two varieties. The first supposes that life came into being in “black smokers”, hydrothermal vents formed along undersea ridges such as the Mid-Atlantic Ridge. Sea water leaks down through fissures in the rock and is super-heated by magma. The super-heated water may attain a temperature of 400°C, but the immense pressure keeps it from boiling. When the mineral-laden water rises and hits the relatively colder sea water, dissolved minerals are liberated, emitting sulfur-bearing black molecules which look like, but are not, smoke and which pile up to produce “chimneys”. White smokers, which carry barium, calcium and silicon, also exist. It was thought that the reaction of hydrogen sulfide from the vent with water would provide the energy necessary for the formation of life. Although life does abound in these vents, it is not at all like ours. One finds, for instance, extremophile organisms which live in darkness and obtain their nourishment from hydrogen sulfide.
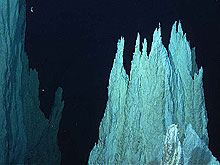
“Nature Tower”, an alkaline “chimney” in the Lost City group. From NOAA.
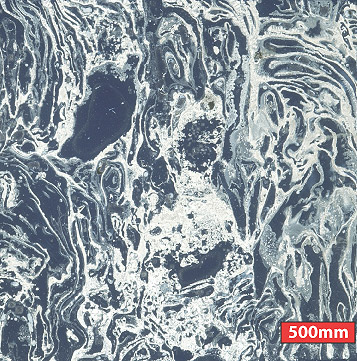
Whorls and pores in a thin section of a Lost City chimney, from NOAA.
The second hydrothermal-vent model proposes that life originated in alkaline hydrothermal vents. In places on the ocean floor, peridotite rock, which is normally found deep in the Earth’s mantle, has been pushed up to the surface by faulting. The rock contains olivine, which reacts with sea water to form the minerals serpentine and magnetite; the process is called serpentinization, The reactions are exothermic and increase the volume of the reactants. The heat is generated by chemistry and does not come from hot magma, as is the case with “black smokers”. The result is an alkaline solution (pH = 9-11) rich in calcium and H2. The rocks produced have lower density and so expand and push up. They crack and more sea water moves in to react with remaining olivine. On contact with colder sea water, the calcium precipitates out, forming white structures like chimneys. Eventually, small cracks and “cells” form within the rock. Rising fluids are very alkaline (basic) and thereby precipitate out calcium carbonate and other alkaline substances when they hit the cold sea water. These then build up on the pile of rock already started and soon “reverse stalactites” are produced by the carbonate left behind by the thermally rising water. Iron in the olivine is oxidized, leading to production of reducing gases hydrogen, methane and hydrogen sulfide. These gases in turn are a source of energy. So the rising “chimneys”, which may reach many meters in height, are associated with a source of energy, gases like those in the “primordial soup” and small cell-sized alveoli or compartments. Such an environment may well be suited to abiotic hydrocarbon production. The compartments contain and protect their contents as well as ensuring their concentration, making excellent conditions for the production of inorganic precursors to organic life. From these, prokaryotes and archea could have evolved independently around 3.8 Gya and eukaryotes later, around 2 Gya.
The volcanic pool hypothesis is more recent. It posits the combination of simple molecular building blocks, perhaps from space, using thermal energy from volcanic pools, like those at Yellowstone or in Iceland. As external conditions change, they could evolve in a Darwinian manner as they survive through wet, dry and moist cycles in land-based hot springs. Such organisms have been called progenotes.
Be that as it may, the appearance of cell membranes meant that different environments and molecules could be separated from each other, a kind of biological differentiation. This led in turn to the the formation of simple cells, called prokaryotic cells.As we have already stated, the earliest clear occurrence of life is in the form of microscopic cells in Archean sediments in Australia, dating from about 3.4 Gya. Cyanobacteria existed by 2.22. Gya and are still alive all over the globe today. The “cyan” in their name refers to their blue-green color. They are the oldest currently-living beings. But they are extremely important for another reason.
Some of these bacteria mixed with sand to make microbial mats. As the sandy mixture became muddy, the cyanobacteria migrated upwards and the process repeated, resulting in lumpy layers of colonies called stromatolites. Stromatolites thrived over the period from about 3.5 Gya to 0.5 Gya, but are still found in a few places such as Shark Bay, Australia, or the Pacific Coast of Baja California. They survive only in especially salty water (twice the sea’s normal saltiness) or in places with especially strong currents, as both conditions limit predators such as snails which otherwise would devour them.
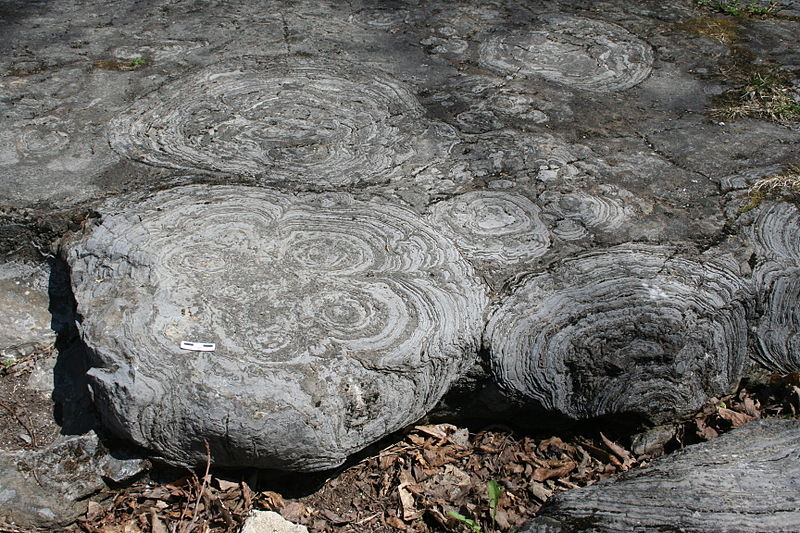
Stromatolites in limestone near Saratoga Springs, NY, by M. C. Ryget via Wikimedia Commons
In addition to that, phylogenetic studies show that eukaryotes form, usually, five supergroups, all of which evolved from a common eukaryotic ancestor. The members of each group have then evolved independently of the other groups. Only eukaryotes have evolved to form complex life and it all conserves properties of the common ancestor. Eukaryote cells are all very similar, all of them having, for example, common methods of cellular respiration, sex and DNA contained in nuclei.
Cyanobacteria have been called the “working-class heroes of the Precambrian Earth” and were fundamental to the development of life. The importance of these organisms cannot be stressed too much, as they were the first organisms to carry out photosynthesis, the use of energy from the sun to convert carbon dioxide into nutrients and free oxygen, which is returned to the atmosphere. Over hundreds of millions of years during the Archean and Proterozoic Eons, as cyanobacteria used photosynthesis to recover the energy necessary for their own metabolism, they brought about the gradual transformation of atmospheric CO2 into the oxygen necessary for other forms of life, such as ourselves. At the same time, the greenhouse effect was reduced and, thereby, global temperatures. Much CO2 was also dissolved in the seas, where it combined with calcium to form calcium carbonate, which in turn solidified to form limestone. Limestone, ocean water and corals are huge stores of carbon dioxide (carbon sequestration).
Interestingly, thousands of the minerals found on Earth today are due to oxidation by oxygen dissolved in water. So oxygen has not only allowed life to begin, but has also thoroughly changed our mineral environment. In other words, the biosphere and the geosphere have evolved together.
Photosynthesis took place in the top layer of stromatolites and each layer lived off the layer above. As such, they represented an early symbiosis or way of living together – an example of what we now call ecology. Notice that ecology (water, atmosphere) led to biology (stromatolites), which in turn influenced ecology (atmospheric oxygen).
The Proterozoic Eon — the dance of the continents
The Proterozoic Eon runs from 2.5 Gy to 542 Mya. It has been so named because of the appearance of more complex organisms during this period,
Geology and atmosphere
In spite of widespread glaciation early on. During this eon, plate tectonics came into its own, with cratons moving about on the surface of the Earth in what has been called a “stately dance”, i.e., a slow one. It was like some kind of round, with one continent dancing for a while with another, then separately, then with a third. At least five times, they all came together to form a single supercontinent. As they smashed into each other, they brought about the rise of mountains, a process geologists call orogeny. As they rifted and came apart, seas formed between them.
There is rather weak evidence for a perhaps small-continent-sized landmass dubbed Vaalbara 3.3 Gya. It is much more certain that there existed a continent-sized landmass called Ur about 3.1 Gya, made up of cratons from what now are South Africa, Australia, India and Madagascar. Ur lasted for about 300 Gy, undergoing various combinations with other continents, until the breakup of Pangea. Ur was not a supercontinent, but it’s about all there was.
It should be remembered that these reconstructions of ancient cratons or continents from geological and other data are to varying extents uncertain as to the details.
About 2.7 Gya, the first supercontinent came into being – Kenorland (also called Superia). Since the atmosphere was devoid of oxygen at the time, only acid rain fell, and this eroded and dissolved the land, leading to the deposit of sediments along the continent’s coasts. About 2.4 Gya, just as oxygen started accumulating in the atmosphere, Ur broke away and Kenorland began its fragmentation.
By 2 Gya, there were at least five separate cratons:
- the Laurentian supercraton, the geological core of North America;
- Ur, composing current India, western Australia and South Africa;
- Baltica and Ukrainian cratons, making up eastern Europe;
- cratons comprising most of what are now South America, China and Africa.
By about 1.8 Gya, all these cratons had collided and coalesced to form the supercontinent Columbia. Since it was situated on the equator, its interior was hot and dry. There were therefore no or few ice caps and ocean levels were relatively high.
Around 1.6 Gya, Ur split off from Columbia and a new sea formed between them. Since they were still at the equator, ice remained low and ocean levels high.
About 1.2 Gya, a new supercontinent now called Rodinia was forming, again near the equator, so its interior was again hot, dry and lifeless and no sediments were formed. Evidence for Rodinia comes from the so-called Grenville orogeny, rocks of which are found in the cratons of all current continents. Also, the absence of sedimentary rocks from this period suggests an absence of shallow seas, which would have been the case if there was only one supercontinent. Rodinia now was surrounded by a single superocean called Mirovia. About 850 to 800 Mya Rodinia broke apart and then, somewhere around 700 Mya, it may have reformed with the pieces in a different order to form another supercontinent, Pannotia, which only lasted about 60 million years before It broke up in term.
![Reconstruction of the supercontinent Rodinia, by John Goodge [Public domain], via Wikimedia Commons](http://natural-universe.net/wp-content/uploads/2015/07/Rodinia_reconstruction.jpg)
Reconstruction of the supercontinent Rodinia, by John Goodge [Public domain], via Wikimedia Commons
The next supercontinent,
Pangea, formed only later, in the Phanerozoic Eon.
It was during the Proterozoic and beginning of the Phanerozoic Eons that the oxygen content of the Earth’s atmosphere began to increase significantly. Alternating layers of red, iron-containing minerals and silica minerals called banded iron formations (or BIFs) indicate fluctuations in the oxygen levels of oceans about 2.5-1.8 Gya, at least not before 1.8 Gya. Iron(II), or Fe2+, is soluble in water, but is oxidized by atmospheric oxygen to iron(III), or Fe3+, which precipitates. Since BIFs exist in sedimentary rocks, it is thought than fluctuating levels of oxygen in the sea water led to the alternating bands of minerals.
Later formations called red beds, which are sedimentary sandstone or shale, exist from 1,8 Gya. Their red color is due to the mineral hematite, Fe2O3, formed by the oxidation of iron, but this time on land. So by this time, the air must have contained enough oxygen to oxidize iron. Red beds are also common in rocks from the Phanerozoic Eon.
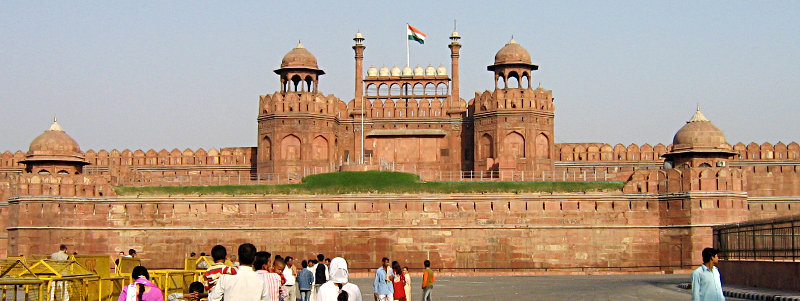
The Lal Qila, or Red Fort, in Delhi is built of red-bed sandstone. Photo by Siv O’Neall.
In summary:
- From 2.5-1.8 Gya, fluctuating oxygen content in seawater formed BIFs.
- Since 1.8 Gya, increasing atmospheric oxygen has oxidized Fe to hematite.
Atmospheric oxygen also allowed the formation of new types of minerals, so once more life influenced geology.
Life
Once the great oxidation event had taken place, life now went through a long, slow period often referred to as the boring billion. Nevertheless, it included the oxidation of the atmosphere and evolution of eukaryotes.
Evolution and the atmosphere
As shown by fossil evidence, stromatolites thrived in the Proterozoic and continued their conversion of atmospheric CO2 into O2. The first oxygen produced had been gobbled up by chemical reactions like the oxidation of iron. Several types of indirect evidence, based on the presence of certain molecules in rocks, indicate that around 2 Gya, the content of free oxygen in the atmosphere increased significantly. It is generally accepted that this increase began about 2.4 Gya in what is called the Great Oxidation Event. In spite of evidence for important fluctuations in oxygen levels over the millenia since then, the average oxygen content of the atmosphere has been increasing for the last two billion years. It is now at about 21%, a figure to be compared with less than 1% at the beginning of the Proterozoic.
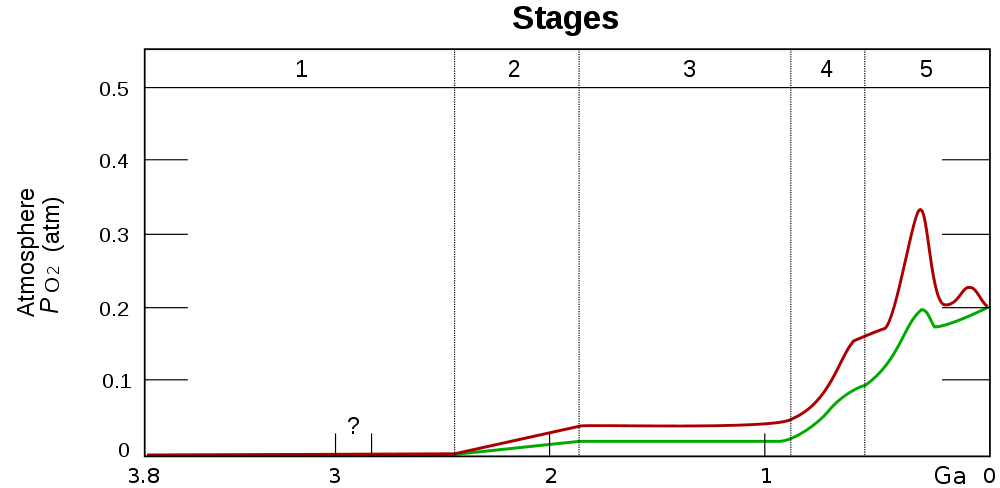
Estimated evolution of atmospheric O2 percentage, by Heinrich D. Holland via Wikimedia Commons. The red and green lines are ranges of estimates.
With the atmosphere richer in oxygen, other forms of life evolved. More complex cells called eukaryotes appeared about 1.4 Gya. Such cells incorporate smaller components called organelles. Examples are the cell nucleus and the mitochondria essential to the generation of energy for the cell. It is now widely accepted that organelles within eukaryotes were bacteria which entered the original cell, be it prokaryote or some sort of proto-eukaryote, and stayed – a process referred to as endosymbiosis.
Prokaryotes reproduce by a process of mitosis, duplication and division, after which each “child” organism is essentially a clone of the “parent”. Eukaryotes also duplicate themselves by mitosis, but they reproduce by meiosis, a process in which a selection of genes from each parent is combined with a selection from the other. This method of reproduction leads more rapidly to greater diversity of genes and, so, to the formation of new species. Only eukaryotes form multicellular organisms, a necessity for more advanced forms of life.
Taking into account biochemistry and evolutionary history, biologists now usuall divide life into three domains: bacteria and archaea, (both prokaryotes), and eukarya, the last two being descended from the first in a yet-to-be-agreed-on order. Current eukarya include plants and animals – such as us. One proposed Tree of LIfe is shown below.
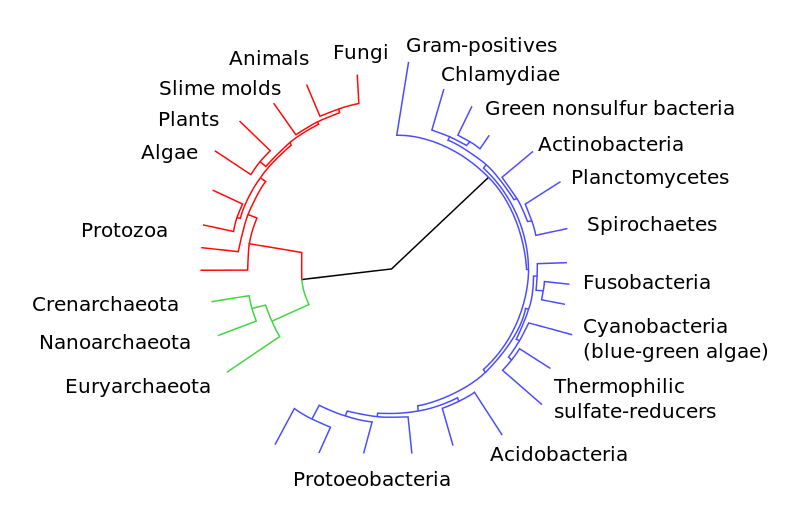
Tree of Life. Eukaryotes are colored red, archaea green and bacteria blue. From Wikimedia Commons
Such trees of life depend on comparisons of certain genes across species and the choice of genes has an influence on the resulting tree. So this is one among many. It is also argued that life forms not a tree, but a network, or mesh.
In addition to that, phylogenetic studies show that eukaryotes form, usually, five supergroups, all of which evolved from a common eukaryotic ancestor. The members of each group have then evolved independently of the other groups. Only eukaryotes have evolved to form complex life and they all conserve properties of the common ancestor. Eukaryote cells are all very similar, all of them having, for example, common methods of cellular respiration, sex and DNA contained in nuclei.
Climate instability and glaciations
The Earth’s climate now entered a period of great instability. The initial cause may have been imbalances in the geosphere and biosphere. The period of existence of a single continent, Rodinia (or Pannotia), surrounded by a single ocean under an atmosphere still low in oxygen was coming to an end around 750 Mya. New coasts brought more shallow coastal seas and bays which in turn allowed more algal blooms. These may have gobbled up CO2 as did rock weathering, leading to a global cooling.
Whatever may have been the cause, Earth now embarked on an instable period of glaciations referred to as Snowball Earth, although “slushball” might be a better term. Evidence for glaciers between 740 and 580 Mya ago comes from all around the Earth. Life survived, probably in warm, underwater hydrothermal vents. Eventually, CO2 pumped into the atmosphere and methane, CH4, perhaps manufactured by methanogen bacteria, conspired with other feedback effects to bring about global warming and end the glaciations. Then it started all over again. Over 150 million years, at least three cycles of ice age followed by global warming occurred.
- The Sturtian glaciation peaked about 720 Mya;
- the Marinoan glaciation, about 650 Mya; and
- the Gaskiers glaciation, about 580 Mya.
Characteristic rocks left behind by retreating glaciers attest to these cycles of cold and hot. Between the second and third cycles, oxygen levels reached levels near those of today and animal life took off.
Ediacaran fossils
Fossils usually only show the harder body parts of the fossilized organisms. But from the end of the Proterozoic, around 575-542 Mya, fossils were discovered which also showed the softer body parts of strange and complex organisms. Named after the Ediacaran Valley in Australia where they were first discovered, they have since been found around the world in places such as Charnwood Forest, England, or Mistaken Point, Newfoundland.
The Ediacaran fossils are difficult to interpret. They seem to be generally flat, multi-sectioned organisms, often described as “quilted”, without any internal structure. Charnia, for instance, seems to be a flat, fractal construction without any central stalk. They do not resemble any modern organisms and are generally considered to represent an evolutionary dead end in spite of their being complex, multi-celled organisms. In any case, since they date from as much as 575 Mya, they do show that multi-cellular life existed before the Cambrian. After the Ediacarans had lived alone for up to 90 million years, they disappeared forever as small shelled organisms and trilobites took over.
The Phanerozoic Eon – rise of complex organisms
The Phanerozoic Eon is divided into three eras:
- the Paleozoic (542-251 Mya),
- the Mesozoic (251-65.5 Mya) and
- the Cenozoic (65.5 Mya to today… about).
The Paleozoic Era
During the Paleozoic, the buildup of cratons and mountains continued; glaciers and shallow seas were formed. Life spread from the sea to occupy the land; and fishes, reptiles and primitive mammals evolved.
Geologists have found a huge increase in the number, variety and, especially, the complexity of fossils dating from around 542 Mya in western Canada and in China. This date has therefore been adopted as the beginning of the Paleozoic Era, which is considered to run from 542 to 250 Mya. It is itself broken down into six subdivisions called periods, named as follows:
- Cambrian (542-500 Mya),
- Ordovician (500-440 Mya),
- Silurian (440-410 Mya),
- Devonian (410-360 Mya),
- Carboniferous (360-290 Mya) and
- Permian (290-250 Mya).
Geology
Around the beginning of the Paleozoic, as tectonic plates continued moving, Rodinia broke up into Gondwana and Laurentia. About 300 Mya, the sea between them shrank and they collided to form the supercontinent, Pangea. The superocean surrounding it is called Panthalassa. For once, a supercontinent was not located right at the equator; about ¾ of Pangea was in the southern hemisphere.
Life in the sea
The extraordinary increase in the number of multi-cellular animal phyla which took place at the beginning of the Paleozoic has been referred to as the Cambrian Explosion. It is seen today especially as an explosion of fossils. In fact, the word “explosion” is an exaggeration which has led at least one scientist to react and call it the Cambrian “slow fuse”.
For 2 billion years after the appearance of life on Earth before or around 3.5 Gya, only single-celled prokaryotes existed, cyanobacteria diligently working to increase the oxygen content of the atmosphere. Then the enigmatic fossils of the Ediacaran fauna show that multi-celled, invertebrate organisms came and, it seems, went between about 600 and 545 Mya.
The next logical step, the development of some sort of skeleton or carapace, came about in the early Cambrian, about 545-520 Mya, in the form of “small shelly fossils” (SSFs), or just “little shellies”. These tiny creatures had shells of calcium phosphate, presumably because atmospheric conditions did not yet favor the calcium carbonate shells of today. So for about 25 My, the so-called Cambrian Explosion was represented simply by small shelled creatures – not much of an explosion!
Somewhat later, extraordinary fossils including soft parts of the animals were deposited in two remarkable sites. The first one was Chengjiang, China, with fossils dating around 515 Mya. Among the Chengjiang finds is the oldest fish, which is also the oldest vertebrate, dating from about 500 Mya.
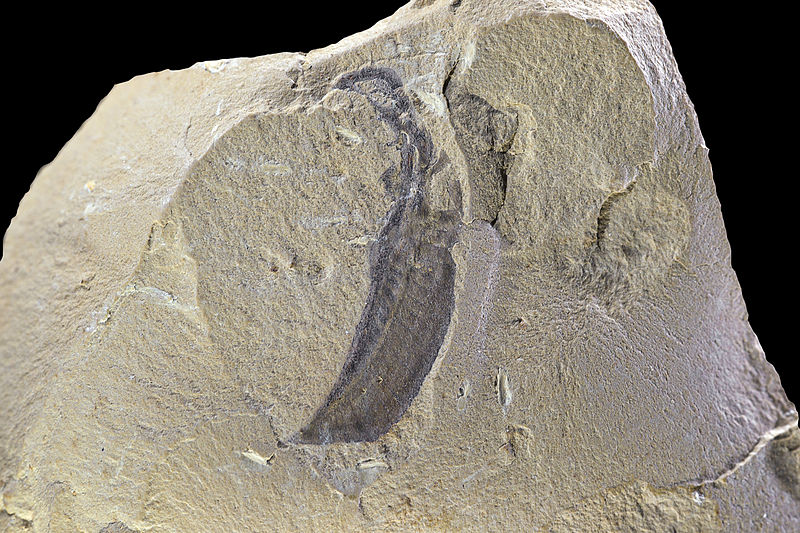
Haikouella lanceolata, from the Chengjian fossils, by Didier Descouens via Wikimedia Commons
Probably the most famous of the Cambrian fossils are those of the Burgess Shale field of about 505 Mya (Middle Cambrian), now in Canada. Some of them were pretty strange and are still the subject of study and hypotheses.
Sponges, considered to be the most primitive animals alive today, had appeared in the late Vendian (end of the Protozoic).
In the early Cambrian, radially symmetry echinoderms were the ancestors of today’s starfish and sea urchins. From about 530 Mya, other invertebrates like brachiopods and worms started to leave fossil traces. Brachiopods, which were shellfish with hard upper and lower valves (as opposed to the left and right valves of modern oysters and scallops, to mention the most edible of them), grew wild on the sea floors.
About 520 Mya, trilobites appeared and invertebrate, multi-celled life was off and running.
Although there do exist fossil tracks of mostly worm-like creatures from 555 Mya, the organisms represented by the Cambrian-period fossils were of a new kind. Cambrian organisms grew to be larger and more complex because of their support structure. During the early Paleozoic, continents were under shallow seas for periods of several million years at a time, so life was dominated by creatures of the seas, including reef builders. These organisms had no internal skeletons, meaning they were invertebrates, but they did have a hard exoskeleton or carapace. The support this gave was advantageous in several ways: It shielded them from the sun, allowed them to retain moisture, gave support for a muscle system and protected them to some extent from predators. Later, skeletons would provide a mineral store, since bones store minerals like calcium and phosphorus from the blood and are able to pass them back to body cells when they are needed. Many types of these creatures existed in the Paleozoic seas. From tiny creatures, larger ones evolved.
Trilobites, a type of arthropod, became a dominant form of marine life. They existed in thousands of different species on every continent for some 270 million years, so long that they have been referred to as the “mascots” of the Paleozoic. They ranged in size from several millimeters to over 50 centimeters. Some had eyes with many crystalline lenses, like fly eyes. Over time thousands of species of trilobites existed – in shallow seas on every continent. Near the end of the Cambrian, there were three trilobite mass extinctions due to climate change and other factors (continental movements, evolution of predators). But trilobites survived.
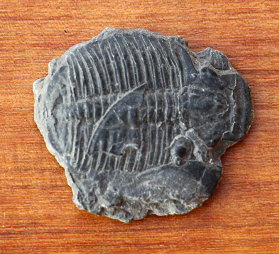
Small trilobite, 5cm (Ohio), photo by author.
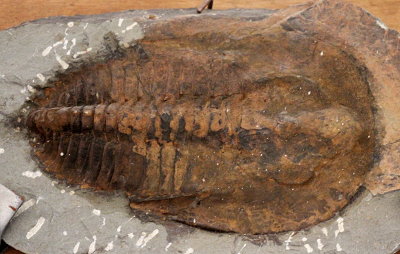
Larger trilobite, ~40 cm (Lourinha, Portugal), photo by author
At the end of the Ordovician and the beginning of the Silurian, two mass extinctions took place, separated by around 4 million years. They are referred to as the Ordovician-Silurian extinction events. Since most life was in the sea, it was this sea life which suffered, It is estimated that 60% of marine invertebrates were destroyed. The extinctions were probably largely caused by climate change due to movement of the continents.
In the Silurian period, eurypterids (looking like scorpions or crayfish) developed which were capable of living in salt or fresh water, an important step in animal evolution. The ammonoids and nautiloids whose fossils we find so beautiful appeared toward the end of the Paleozoic.
The first fossil evidence of fishes show species which had spinal cords (making them chordates) but no internal skeletons or jaws. The latter evolved from gills only later. Fish became numerous in the Devonian Period, which is often referred to as the “Age of fishes”. Although many types later became extinct, some of their ancestors survive even today: cartilaginous fish, like sharks or rays; fish with bones, like today’s trout or bass; and lobe-finned fish, like today’s lungfish.
At the end of the Devonian, another series of extinctions referred to collectively as the Late Devonian mass extinction took place. Individual events may have been separated by over millions of years. Mostly marine life was affected and trilobites were almost finished off.
Life on land
Plants first developed in water. The date of their migration onto land is still debated but seems to have taken place at least by around 480 Mya and perhaps as early as 600 Mya, in the late Precambrian. Low mossy plants appeared on land during the Ordovician. The migration of plants to land was facilitated by the development of a cellulose-based support structure and the ability to transport water in their stems. The oldest known such vascular plant dates from the mid-Silurian, about 430 Mya, and represents an important advance, as such plants had internal tubes by which water and nutrients could mount from the soil to replace moisture that was eliminated from the plant’s upper parts.
With the advent of woody stems, plants developed to the point where the Carboniferous Period was one of dense areas of vegetation, tree-like plants and swamps. Carboniferous plants were all seedless and so had no flowers. This plant material decayed and was eventually transformed by heat and pressure into the fossil fuels we are busily burning up in a tiny fraction of the time it took to make them.
Such carbon sequestration lead to higher oxygen levels in the atmosphere. The oxygen content of the Carboniferous atmosphere was 50-100% greater than now (as seen in a preceding figure) and this had an effect on evolution. Giant insects evolved, including a dragonfly with a 65 cm wingspan. Later, when oxygen levels came back down, the giant insects disappeared.
During the Silurian, tiny arthropods appeared on land. They did not have a digestive system capable of making them herbivores, but lived off decayed matter. During the Devonian, skeletal changes which permitted animals to support themselves on land facilitated the transition from fishes to tetrapods (four-limbed animals, including birds). The first land-based tetrapods were still aquatic or amphibious animals and probably lived mainly in ponds. But they were capable of breathing air, so they could move to another pond in times of drought. They also laid their eggs in water, which furnished nutrients for the young, which were essentially fish (like tadpoles).
So first plants moved onto the land. They were followed by small arthropods, which ate decayed matter from the plants. And then tetrapods followed and ate plants and arthropods. It is all about getting enough to eat.
A very important evolutionary step was the development of the amniotic egg. This protected the young inside a protective cover and provided the nutrients that young amphibians could only get from water. This development contributed greatly to the evolution of amniotes (the first of which resembled small lizards), which now could leave the water completely. These animals split into two groups, synapsids (early mammals) and sauropsids (early reptiles). The first reptiles date from the mid-Carboniferous, during which life on land and sea reached a new peak of development and diversity.
Tectonically, what today would be Europe and North America were then situated in tropical climates near the equator. Indeed, because no land mass was over either pole, polar ice caps were limited and the Earth’s temperature gradient was less pronounced. On land, huge tree-like plants grew in swamps and life reached all the continents. Insects and tetrapods swarmed through the undergrowth. But no bird sang and no flower lent color to the scene.
Near the end of the Carboniferous, as Gondwana (the southern continent comprising today’s South Africa, South America, Antarctica, Australia and India) approached the poles, as seen in a preceding figure, there was a period of glaciation which lasted into the Permian. Remaining glacial features on these continents provide evidence for plate tectonics, as some of these continents now occupy much warmer latitudes.
The Permian Period was dominated by the existence of the supercontinent Pangea. Around the equator, the Carboniferous swamps had given way to deserts and these arid conditions were well suited to the development of reptiles.
To the east, projecting into the continental land mass, was the Tethys Sea, which was swarming with life. This was also true of the Zechstein Sea in the north, the area of current northern Europe. Parts of the Zechstein evaporated, leaving behind minerals (evaporites) which helped furnish raw materials for the Industrial Revolution – plaster of Paris, gypsum and substances used for the production of acids and ammonia.
The distribution and variety of organisms today is a result of the existence and subsequent breakup of Pangea. During its existence, no waterways blocked migration routes, so animals, at least those who could support the aridity of the interior, were free to move about to new habitats. The later breakup of Pangea was an equal boon to evolution as organisms isolated from one another tend to evolve in different ways from similar beginnings. Simply put, “isolation begets diversity.”
The time of Pangea was one of much development in the forms of life. By its end, dinosaurs and early mammals had developed. Many insects existed, including cockroaches, which are still with us, alas.
The end-Permian extinction, among others
The Paleozoic Era ended with the greatest of all the mass extinctions, the end-Permian extinction (or Permian-Triassic extinction), sometimes referred to as the Great Dying. It is estimated that 96% of sea and 70% of land species disappeared. The date of the extinction marks the end of the Paleozoic and the beginning of the Mesozoic Era, largely accepted as 251 Mya.
Studies of the Cretaceous Period have discovered several possible ways in which geology can influence life – especially negatively:
- Intense volcanic activity at various times and places has produced immense quantities of flowing lava called flood basalts, for which geologists have coined the acronym LIPs (large igneous provinces). LIPs were formed quickly on the geological timescale, in less than a million years, but may cover up to millions of square kilometers and be several km thick. They are thought to have formed over deep plumes of magma, much like the Hawaiian Islands, and so can form on land or under seas, independently of plate boundaries.
- Black shales are darkly colored Cretaceous rocks rich in carbon which form when large amounts of plant and animal life die and descend to the sea floor. The shales only can form when the deep water contains no or almost no oxygen. The periods when such conditions hold are referred to as oceanic anoxic events, or OAEs. OAEs have been discovered worldwide, in all oceans and on land. They arise and disappear abruptly and have been shown to be associated with times of global environmental change. Their tendancy to occur at the same time as LIPs is circumstantial evidence associating the two phenomena.
- Isotope ratios of osmium in sedimentary rocks depend on whether the sediment comes from continental rocks or sea-floor volcanic activity. The data show that during periods of black-shale production osmium in sediments comes almost all from underwater volcanoes and not from continental weathering. So LIPs and OAEs seem to occur at the same time as strong sea-floor volcanic activity.
Although these findings concern the Cretaceous period, they suggest strongly that LIPs have had significant effects on global climate, mainly originating in the emission of large quantities of carbon into the atmosphere, disrupting the carbon cycle.
Other suggested causes are the following.
- So-called green sulfur bacteria live in the ocean by photosynthesis and by consumption of sulfur dioxide. Biomarkers for these bacteria therefore indicate the presence of sulfur dioxide. Their presence at the same time as OAEs suggests that some of the extinctions may have been due to this toxic gas.
- A recent study indicates that the eruptions in the Siberian Traps increased the amount of nickel in the Earth’s crust and this was a nutrient for a microbe, a methane-producing archaea called Methanosarcina, which had undergone a genetic change at about that time. It is suggested (claimed, even) that the microbe emitted vast amounts of methane into the atmosphere and so changed the climate.
So much for the details. The important finding based on the importance of LIPs for global change is the discovery of three major LIPs which occurred at the same time as three major mass extinctions:
- The Siberian flood basalts, or Siberian traps, occurred around 252 Mya – at the time of the end-Permian extinction.
- The Central Atlantic Magnetic Province (CAMP) occurred about 200 Mya – at the time of the Triassic-Jurassic mass extinctiona.
- The Deccan traps in India were formed about 65 Mya at the time of the K-T extinction.
So the end-Permian extinction was probably initiated by volcanic eruptions in Siberia which increased the amount of methane and CO2 in the atmosphere, disrupting the carbon cycle and bringing about a “runaway greenhouse phenomenon” . This in turn would have caused oceans to release dissolved oxygen. It could have caused acid rain which killed land plants vital to survival of animals. The CO2 would have been absorbed by the oceans and led to their acidification, wiping out many marine organisms.
What is clear is that it took around 20 million years for life to recover, far longer than after the other known mass extinctions. When life did attain its previous diversity, its forms had changed. The few remaining trilobites had been completely eliminated.
In spite of a wealth of possible explanations, there is yet to be a clear consensus as to which are correct.
Don’t stop now. Continue global history with the ages of reptiles and mammals.