Memory is stored in the brain by means of neural connections (circuits) and so is based on synaptic weights – relative strengths of synapses. These are not programmed in our genes, as there are not nearly enough genes to contain such an immense recipe. Instead, synaptic enhancement is due to correlated activity brought about by chemical substances between the pre-synaptic and post-synaptic elements. This method has the advantage of allowing environmental influence on learning.
It hardly needs to be pointed out that memory is essential not just to what we are, but to our very survival. The “present” we inhabit is really a collection of memories, some from the last milliseconds (what we see as “present’), filled out by and compared to memories of similar past experiences. It really is the “remembered present”.1Edelman (2006).
Types of learning and memory
Neuroscientists and psychologists distinguish several types of memory, which have different functions and are handled and stored differently in the brain.
- Explicit or declarative memory is conscious. It starts out as short-term memory in the pre-frontal cortex and may later be stored as long-term memory via the hippocampus. Explicit memory in turn is divided into two parts.
- Semantic memory refers to remembered facts.
- Episodic memory refers to remembered experiences, events and episodes.
- Implicit or procedural memory is unconscious. It contains what is necessary for all those procedures we do without thinking, like riding a bike or driving a car, playing a musical instrument or tennis. It is in fact a collection of different systems and depends on the amygdala (emotional responses), the cerebellum (motor control) or the striatum, a part of the “reward system” and input to the basal ganglia (procedural memory).
In addition, memory can be short-term, one type of which is working memory, or long-term.
Scientist also study several basic types of implicit or procedural learning.
- Non-associative learning describes memory due to a single stimulus. The two types of non-associative memory are:
- Habituation is just that, getting used to a stimulus (such as background noise) so you stop paying attention to it.
- Sensitization is the opposite of habituation, like “getting burned” once so any heat frightens you unduly afterwards.
- Associative learning forms associations between two events and also exists in two types.
- Classical conditioning is the association of a neutral stimulus (like a bell ringing) with a real or effective one (the smell of food). The effective response is called the unconditional stimulus (US) because it works all the time; the learned one is called the conditional stimulus (CS). Such conditioning is effective only if the CS occurs at the same time as or slightly, but not too much, before the US.
- Instrumental conditioning is associating a stimulus with a reward, a behavior with a result.
Now let’s look at mechanisms of learning and memory.
Simple implicit learning in invertebrates
Several mechanisms for learning have been identified, all based on the basic fact of learning and memory, which is this:
Neural circuits, the basis of what goes on in the brain such as learning or plasticity, are set up by modification of synaptic strengths.2I see it as the enhanced synapses’ having less resistance to passage of electrochemical neuronal circuits, so that signals take the path of least resistance from one neuron to another. Physicists like minimizing paths.
This synaptic modification depends on correlated activity in pre- and post-synaptic terminals. The idea is conveyed by the popular statement, “Neurons that fire together wire together.”
Consider some examples which give a good idea of the process – or processes – behind learning. They also illustrate what seem to be some common components of all learning.
Short-term-learning in invertebrates
Studies of the giant sea slug, Aplysia, have demonstrated mechanisms for implicit learning.3All the discussion of Aplysia is based on Kandel (2006). A simple stimulus, a puff of air on a part of the animal called a siphon, causes a reflexive retraction of the gill. This is called the gill-withdrawal reflex. After a series of gentle puffs, the animal becomes habituated and no longer retracts the gill as much. Molecular study of habituation has found it to be due to the pre-synaptic terminal, specifically to the production of less Ca++ and therefore reduced release of the neurotransmitter, glutamate, by the synaptic vesicles. Exactly how this happens is not yet known. Nevertheless, the animal has learned that the gentle puff is not worth getting excited over. The effect is short-term, it disappears after a while.
When a series of electric shocks is delivered to the head or tail, the animal retracts the gill quickly upon even a gentle, subsequent puff on the siphon. It has become sensitized, without any simultaneity of the two signals.
This process also has been understood in detail on the molecular level. In sensitization, the axon of the sensitizing or modulating neuron from the tail makes synapses on the cell body and especially on the pre-synaptic axon terminal of the sensory neuron from the siphon at its synapse with the motor neuron to the gill. (So synapses are not always formed between an axon and a dendrite.) The series of tail shocks causes the modulating neuron to release a different neurotransmitter, serotonin, as a first messenger into the synapse between itself and the pre-synaptic terminal of the sensory neuron. The first messenger activates a metabotropic receptor on the sensory axon terminal and this in turn releases a second messenger inside the axon – our old friend cyclic AMP, or cAMP.4We met cAMP when we looked at how the lac operon works. The cAMP activates an enzyme called protein kinase A, or PKA.
Kinases activate a substrate by phosphorylation, adding a phosphate group to it. PKA itself is composed of two regulatory and two catalytic units. The catalytic units do the phosphorylation, but only if the regulatory units have been knocked off by binding with cAMP.
In Aplysia sensitization, PKA phosphorylates a protein which is a K+ channel, causing it to act more slowly. This slows re-polarization of the cell after the peak of an action potential and increases the effect of the sensory synapse. This also allows more Ca++ to enter the cell, causing the release of more glutamate. The effect is sketched in part (b) of the following figure. In addition, more recent research indicates the existence of a post-synaptic effect due to the installation of new glutamate receptors.
The Aplysia also has been the subject of the study of associative learning. In this procedure, a strong shock to the tail (the US) is associated with a gentle puff on the siphon (the CS). It is essential that the CS begin shortly before the US, which will thus come to be predicted by the CS. After repeating this association, a simple puff on the siphon brings about a much stronger response than before, more even than after sensitization. The puff, the CS, brings about an action potential in the cell and this causes release of Ca++. Since the US arrives just after the CS, the already released Ca++ then causes even more cAMP to be produced in response to serotonin released by the US. More cAMP means an enhancement of the synapse which is remembered for a while after conditioning is over. So it is the CS-US coincidence which brings about the increased reaction in this case of associative learning. The CS causes the animal to expect the arrival of the US even when it does not.
We can note four points here:
- This example of simple classical conditioning differs from sensitization only by the synchronization of the CS and US.
- All three forms of short-term learning have served to increase communication between cells.
- All three forms of learning show that already-existing synapses (due to genes and development) are modified by experience (the environment).
- Comparison of sensitization to associative learning shows that basic forms of synaptic modification may be combined into new ones.
A similar mechanism has been found in the fruit fly, Drosophila, suggesting that the same process occurs across species, one more case of evolution’s adopting the same solution in different species.
Since the above reactions are unconscious and weaken with time, they are examples of implicit, short-term learning. They should not be taken as explanations of all cases of learning, as there are other mechanisms which contribute.
Long-term learning in invertebrates
Short-term memory is brought about by local modifications of existing synapses, when second messenger cAMP and PKA enhance glutamate release. Long-term learning, though, is a more …well, long-term affair. This is because it requires addition of new connections which in turn need synthesis of new proteins. This requires DNA expression, which takes place only in the nucleus in the cell body and so is not due only to activity local to the synapse.
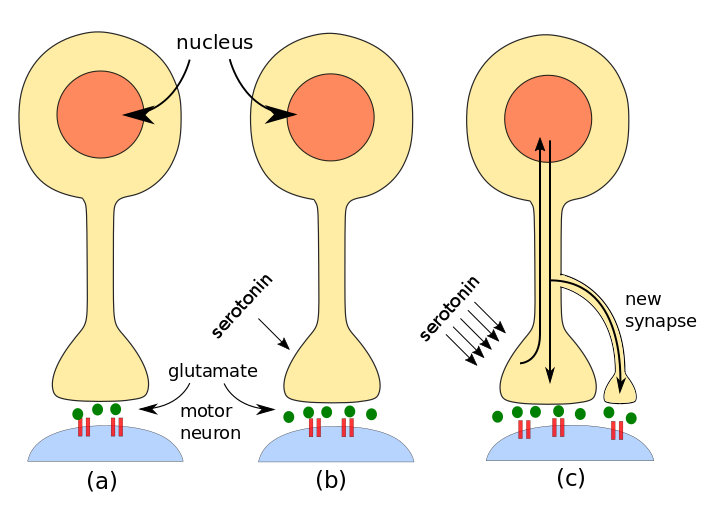
Steps in invertebrate learning: (a) simple stimulus, (b) short-term learning, (c) long-term learning. By author after Kandel.
A series of shocks to the tail of Aplysia brings about long-term learning by the additional formation of more synapses. On the molecular level, the process begins as for short-term memory. A modulating neuron releases serotonin, but more of it than in the short-term case. Not only is PKA activated, but also a second kinase called MAP kinase.5Mitogen-activated protein. Both PKA and MAP move to the neuron’s nucleus in the cell body and activate a regulatory protein (transcription factor) called CREB.6Creb is cyclic AMP response element-binding protein. You see why it’s called CREB. There are two forms of CREB:
- CREB-1 promotes gene expression and is activated by PKA;
- CREB-2 inhibits gene expression and is activated by MAP.
So some genes are activated and some are suppressed in order for the appropriate proteins to be produced by the processes of transcription and translation already studied. The proteins and mRNA then travel back from the nucleus to the axon where they promote long-term synaptic facilitation through the growth of new synapses. But this must only take place on the specific axon terminal, not others, and this requires yet another chemical substance.
A molecule called CPEB7CPEB is cytoplasmic polyadenylation element-binding protein. is found in neurons of Aplysia, Drosophila, mice and humans – and so probably every animal species. It is (or is similar to) a prion, i.e., it exists in two different conformations (shapes). Serotonin causes CPEB to switch from its inactive to its active form. Since the active form is present only in the axon terminal which has been modulated by serotonin, it can serve as a signal to the proteins and mRNA coming from the nucleus which axon terminals they should enhance by making new synapses. Very importantly, CPEB also assures the continuation of local protein synthesis using mRNA from the nucleus, in effect perpetuating the increased synaptic strength. This is long-term implicit learning.
It is also the only known case where the activated form of a prion serves a useful (to us) purpose. Other prions, such as those in mad-cow or Creutzfeldt-Jakob disease, are lethal.
What we have learned about implicit learning in invertebrates: Both short- and long-term memory depend on second messenger cAMP whose synthesis is controlled by serotonin. Whereas short-term implicit learning takes place locally at synapses, long-term memory requires contact with the nucleus and expression of new proteins in order to grow more synapses and reinforce short-term changes.
Learning in vertebrates
A neuron will fire an action potential if its total synaptic input is above a threshold. Normally, a single input will have little or no effect, but a number of inputs can have an effect. If
- an axon tries repeatedly to activate a cell which
- is already strongly activated by another or other inputs at the moment when the input under question arrives,
then the synapse of the first axon with the cell is enhanced in strength.
Similarly, but surprisingly, if the input arrives when the post-synaptic neuron is weakly activated, the synapse of the pre-synaptic neuron is diminished in strength.8The last two statements correspond to what is referred to as Hebb’s law.
Studies of London taxi drivers have shown clearly that the hippocampus is the seat of spatial memory, a type of explicit memory. One way such synaptic strengthening can take place in the hippocampus is through the mechanism of long-term potentiation (LTP), which requires the synchronized firing of NMDA receptors and AMPA receptors on a post-synaptic terminal.9AMPA = α-amino-3-hydroxyl-5-methyl-4-isoxazole proprionate; NMDA = N-methyl-D-aspartate. Although both are glutamate-gated receptors, their function is somewhat different. AMPA receptors allow only Na+ and K+ to cross the cell membrane, whereas NMDA receptors also allow large amounts of Ca++ to enter.
Normally, the channel of the NMDA receptor is blocked by the presence of a Mg++ ion, which keeps the passage of Ca++ minimal. Even if NMDA channels are gated by glutamate, they will remain blocked by the Mg++. Only if AMPA channels first adequately depolarize the target cell will the voltage-gated NMDA channels open. A low-frequency action potential in the pre-synaptic terminal releases only moderate amounts of glutamate, so the post-synaptic AMPA receptors allow in moderate amounts of Na+. However, a high-frequency pre-synaptic action potential releases much more glutamate which in turn causes the AMPA receptors to allow in much more Na+. Electrostatic forces due to the resulting increase in positively charged Na+ in the post-synaptic terminal push out the Mg++ from the NMDA receptors, which then allow large amounts of Na+ and, especially, Ca++ to enter the cell.10Why does the electrostatic potential push out Mg++ but allow Na+ to enter? This only happens if there is a significant amount of Na+ inside the cell and if the NMDA receptor has already bound glutamate outside the cell. So opening of the NMDA receptor requires both pre- and post-synaptic events, making it a coincidence detector and acting like an AND gate.
Once inside the cell, Ca++ acts as a second messenger and brings about a number of results. First, it causes AMPA channels already present in the interior of the cell to be inserted into the membrane of the receptor cell, thus strengthening the synapse.
AMPA receptors recently installed during LTP disappear after a while if no other event takes place. But prolonged Ca++ presence can bring about production of cAMP and PKA which, as in implicit long-term memory, contribute to protein expression by an increase in the CREB transcription factors and synthesis of new AMPA receptors. They also lead to growth factors which can bring about new synapses between the pre-synaptic and post-synaptice neurons., again strengthening the connection.
One can see how this process can lead to associations in neuronal circuits. Suppose a neuron has synapses from three inputs, say, X, Y and Z. If X fires all alone, it is incapable of depolarizing the post-synaptic cell enough for LTP to occur. The same is true for each of the other two synapses. But if both X and Y fire simultaneously, i.e., repeatedly over the same short time period, their cumulative effect may be strong enough to depolarize the cell sufficiently for LTP to take place, meaning that the X and Y synapses will be strenghtened by the means we have seen. Now, if either X or Y brings an input, the post-synaptic neuron may fire, meaning that X or Y have the same result and are therefore associated. The third synapse, Z, will not have been enhanced.11This excellent example is based on one given by Bear et al.
Another possible means of synaptic enhancement may be due to the presence on dendrites of tiny “spines”, some of which have bulbous heads, which has led to their being described as shaped like mushrooms, doorknobs or even punching bags! They are thought to contribute to synaptic plasticity because they have both AMPA and NMDA receptors. They can appear and disappear, and grow and change their shape, depending on activity on both sides of the synapse. They also contain ribosomes, which are normally found near the cell body where they fabricate proteins from the recipes transcribed from DNA by mRNA. Their presence in dendritic spines is thought to be correlated with protein production which may also modulate synaptic strength. Research continues.
Other mechanisms, such as phosphorylation of AMPA receptors, are beyond the scope of this document.
A phenomenon similar to LTP, long-term synaptic depression, or LTD, in which AMPA receptors are removed from the synapse, explains the weakening of synapses in the case of a weakly activated synapse.
Another possible means of synaptic enhancement is due to the presence on dendrites of tiny “spines”, some of which have bulbous heads, which has led to their being described as shaped like mushrooms, doorknobs or even punching bags! They are thought to contribute to synaptic plasticity because they have both AMPA and NMDA receptors. They can appear and disappear, and grow and change their shape, depending on activity on both sides of the synapse. They also contain ribosomes, which are normally found near the cell body where they fabricate proteins from the recipes transcribed from DNA by mRNA. Their presence in dendritic spines is thought to be correlated with protein production which may also modulate synaptic strength. Research continues.
Other mechanisms, such as phosphorylation of AMPA receptors, are beyond the scope of this document.
Similar mechanisms must work during the modification of brain circuity during embryonic development.
We see now that Ca++ is extremely important to the organism for
- formation of bones and teeth
- neurotransmitter secretion in pre-synaptic cells
- muscle contraction
- neural plasticity.
Overview of senses and memory
In brief, the learning process goes something like the following diagram, where we are learning what it is like to stroke a cat on our lap. The diagram shows only a schematic representation of the process, which is in reality much more detailed.
In order to see a greatly simplified version of what takes place in learning, we can regard it as taking place in three steps:
- Perception
- First, we have the cat we are looking at, listening to (if it purrs) and stroking.
- Light and sound waves travel to eyes and ears, somatosensory perception takes place through the fingers.
- Signals produced in the sense organs in the retina (seeing the cat), hands (feeling its fur) and ears (hearing it purr) are transferred to the thalamus. (If the cat had just rolled around in something smelly, which well-brought-up cats do not do, olfactory information would go directly to the olfactory cortex before being projected back to the thalamus and then relayed to the cortex for consciousness.)
- The thalamus routes the information to the appropriate cortex areas (Visual, Somatosensory or Auditory Areas 1 and higher) for analysis. Many scientists think that the combined activity of these areas of cortex is what constitutes the perception of the experience
- Formation of short-term memory
- Projections occur from the neocortex to the lateral prefrontal cortex (LPC), which creates temporary links to them. For our purposes, we may say this set of signals constitutes the image we “perceive” momentarily in our minds.
- The projections from the neocortex sensory areas to the LPC work in both directions. The LPC sends signals back to the cortex, bringing about activation of neurons similar to those evoked by the original image (sight + feel + sound). But this time, rather than originating in the sense organs, the signals are coming directly from the LPC. In this way, the LPC causes the set of neuron activations (the image) to remain in the neocortex longer than it might have done otherwise. This is working memory, one form of short-term memory. Most people are limited to no more than seven items at a time in working memory.12According to my wife, I am limited to only one – maybe two, if you include breathing.
- Remembering
- The hippocampus also is connected to virtually the entire neocortex, as well as the LPC. Like the LPC, it can bring about a set of connections in the neocortex (i.e., alteration of synaptic strengths), which is at the core of thinking about the image in question. Repeated thinking like this is called rehearsal. Over time (maybe several days), such rehearsal, which takes place predominantly during REM sleep13Deep sleep in which dreaming is related to Rapid Eye Movement (REM)., leads to a long-term memory of the event, again, in the same part of the sensory cortex where the original sense-data experience was stored.
Consciousness and all that
To be quite frank, it seems that not a whole lot is really known about consciousness. It is the subject of much conjecture by both scientists and philosophers . Some one of these has suggested that consciousness may be considered as awareness expressed by language. Awareness is related to perception. Clearly, we need some more precise definitions of what all these things are.
We have skipped lots of subjects, perhaps more than in other chapters. Among further considerations which should be taken into account are the following:
- Planning and decision making. Choice – do we have any?
- Language and speech.
- Sleep and waking.
- Emotions, on which subject whole books have been written14Such as those of Antonio Damasio..
- Repair and regeneration in the NS.
Notes
↑1 | Edelman (2006). |
---|---|
↑2 | I see it as the enhanced synapses’ having less resistance to passage of electrochemical neuronal circuits, so that signals take the path of least resistance from one neuron to another. Physicists like minimizing paths. |
↑3 | All the discussion of Aplysia is based on Kandel (2006). |
↑4 | We met cAMP when we looked at how the lac operon works. |
↑5 | Mitogen-activated protein. |
↑6 | Creb is cyclic AMP response element-binding protein. You see why it’s called CREB. |
↑7 | CPEB is cytoplasmic polyadenylation element-binding protein. |
↑8 | The last two statements correspond to what is referred to as Hebb’s law. |
↑9 | AMPA = α-amino-3-hydroxyl-5-methyl-4-isoxazole proprionate; NMDA = N-methyl-D-aspartate. |
↑10 | Why does the electrostatic potential push out Mg++ but allow Na+ to enter? |
↑11 | This excellent example is based on one given by Bear et al. |
↑12 | According to my wife, I am limited to only one – maybe two, if you include breathing. |
↑13 | Deep sleep in which dreaming is related to Rapid Eye Movement (REM). |
↑14 | Such as those of Antonio Damasio. |